Bile acids (BA) are synthesized from cholesterol in the liver and are a significant component of bile. They are stored in the gallbladder and released into the small intestine after a meal. BA are amphipathic steroid acids and are known as indispensable detergents, expediting the digestion and absorption of dietary lipids and lipophilic vitamins by forming micelles in the small intestine. Recently, they have also been considered to be signaling molecules. They are now recognized as ligands of farnesoid X receptor (FXR), a nuclear hormone receptor, and Takeda G-protein receptor 5 (TGR5), a G-protein-coupled receptor (GPCR) that is also known as G protein-coupled bile acid receptor 1 (GPBAR1) [
1].
Bile salt composition of a variety of vertebrate species is validated. Bile salts include C
27 bile alcohols, C
27 bile acids, and C
24 bile acids. C
24 bile acids exist in all vertebrates, although C
27 bile alcohols and C
27 bile acids exist in fish, amphibians, reptiles, and birds [
2]. 5α-C
27 bile alcohol sulfates are considered as the first ancestral bile salts. 5α-cyprinol sulfate is 5α-C
27 bile alcohol sulfate and activates FXR from the frog and the zebrafish, but not from the human and the mouse. In contrast, taurochenodeoxycholic acid (TCDCA) and lithocholic acid (LCA) activate FXR from the human and the mouse, but not from the frog and the zebrafish. Thus, the structure of FXR may be changed to adapt to bind species-specific bile salts [
3].
BA regulate glucose and lipid metabolism by activating these receptors in various peripheral tissues, including the liver, brown and white adipose tissue, skeletal muscle, and the pancreas [
4]. In addition, a recent study has shown that 20 different kinds of BA can be found in the central nervous system (CNS) and the presence of two primary bile acids, chenodeoxycholic acid (CDCA) and cholic acid (CA), in the brain is essential [
5,
6]. In this review, we focus on recent advances in the understanding of the biosynthesis, signaling, and neurological functions of BA.
Synthesis of BA
BA are predominantly produced in the liver, via two biosynthetic pathways: The classical (or neutral) pathway and the alternative (or acidic) pathway [7] (). The synthesis of BA from cholesterol involves at least 16 enzymes [8]. The classical pathway is initiated by cytochrome P450 7A1 (CYP7A1), also known as cholesterol 7α hydroxylase. Cholesterol is converted to 7α-hydroxycholesterol by CYP7A1, which is the rate-limiting enzyme in this pathway. 7α-hydroxycholesterol is then converted to 7α-Hydroxy-4-cholesten-3-one. Cytochrome P450 8B1 (CYP8B1), also known as sterol 12α-hydroxylase, is responsible for the production of CA from 7α-hydroxy-4-cholesten-3-one, but 7α-hydroxy-4-cholesten-3-one is also converted to CDCA by cytochrome P450 27A1 (CYP27A1), also known as sterol 27-hydroxylase. The alternative pathway begins with the conversion of cholesterol to (25R)-26-hydroxycholesterol [9] by CYP27A1. Cytochrome P450 7B1 (CYP7B1), also known as oxysterol 7-α-hydroxylase, leads (25R)-26-hydroxycholesterol to CDCA. CDCA is converted to α-muricholic acid (MCA), and β-MCA. These BA are then conjugated with glycine or taurine. BA synthesized in the liver are referred to as “primary BA” [10,11,12]. Primary BA in rodents are also conjugated with taurine in the liver [13]. Bile salt export pump (BSEP) and multidrug resistance-associated protein 2 (MRP2) are the transporters that mediate the secretion of BA from hepatocytes into hepatic bile canaliculi (). BSEP is the main transporter of BA, whereas MRP2 principally transports BA glucuronides and sulfates [1,14].
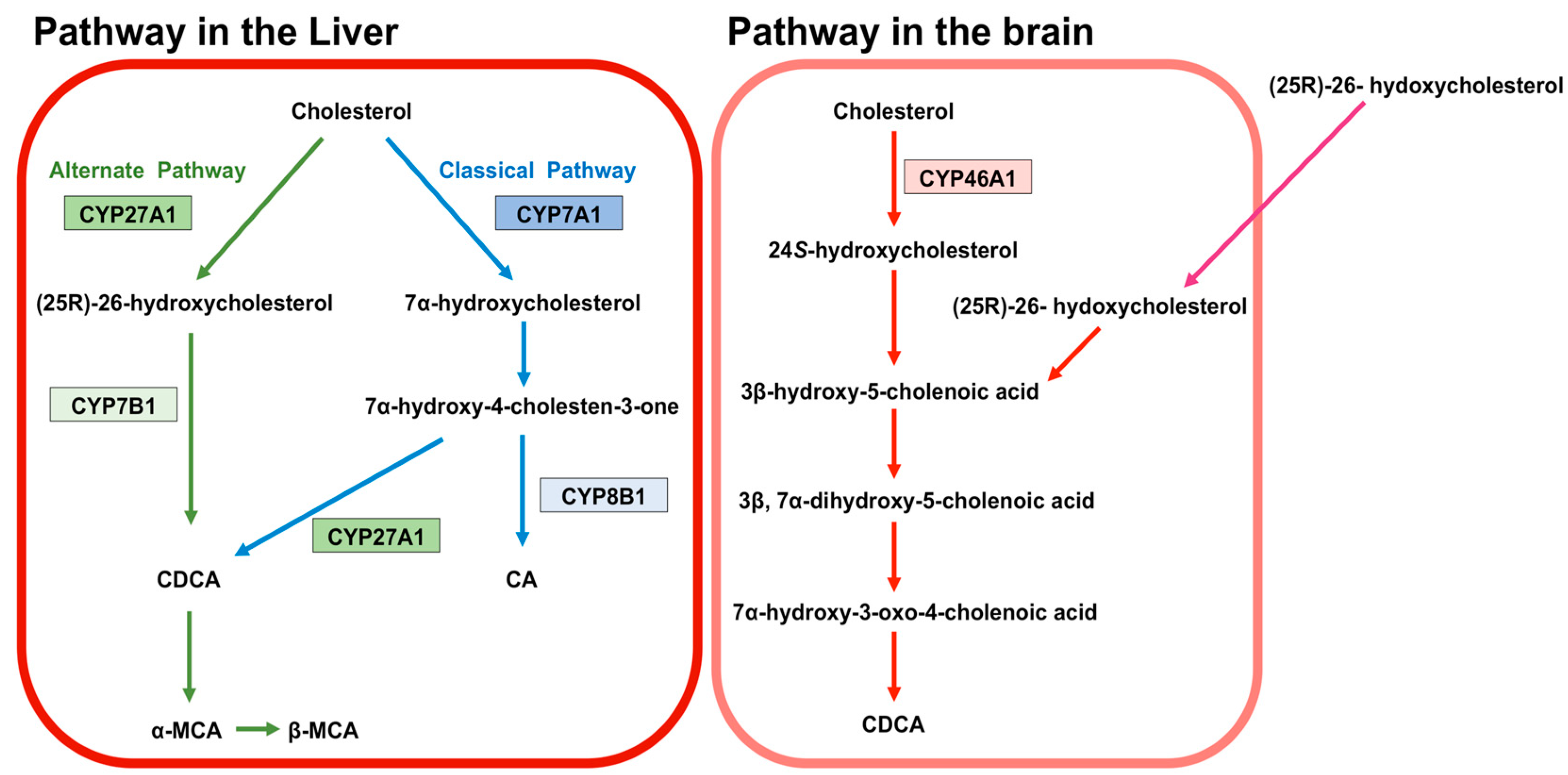
Figure 1. Synthesis of bile acids (BA). The classical pathway is initiated by cytochrome P450 7A1 (CYP7A1). CYP7A1 converts cholesterol to 7α-hydroxycholesterol. 7α-hydroxycholesterol is then converted to 7α-hydroxy-4-cholesten-3-one. Cytochrome P450 8B1 (CYP8B1) leads the production of CA from 7α-hydroxy-4-cholesten-3-one. 7α-hydroxy-4-cholesten-3-one is also converted to CDCA by cytochrome P450 27A1 (CYP27A1). The alternative pathway begins with converting cholesterol to (25R)-26-hydroxycholesterol by CYP27A1. Cytochrome P450 7B1 (CYP7B1) leads (25R)-26-hydroxycholesterol to CDCA. CDCA is converted to α-muricholic acid (MCA), and β-MCA. These BA are then conjugated with glycine or taurine. BA in rodents are also conjugated with taurine in the liver. BA synthesized in the liver are called primary BA. In the brain, 24S-hydroxycholesterol is converted from cholesterol by cytochrome P450 46A1 (CYP46A1). 24S-hydroxycholesterol is a precursor of 3β-hydroxy-5-cholenoic acid, which can be converted to CDCA through the intermediates (3β, 7α-dihydroxy-5-cholenoic acid and 7α-hydroxy-3-oxo-4-cholenoic acid). A large amount of (25R)-26-hydoxycholesterol incorporates to brain from circulation, and (25R)-26-hydoxycholesterol can also be converted to 3β-hydroxy-5-cholenoic acid.
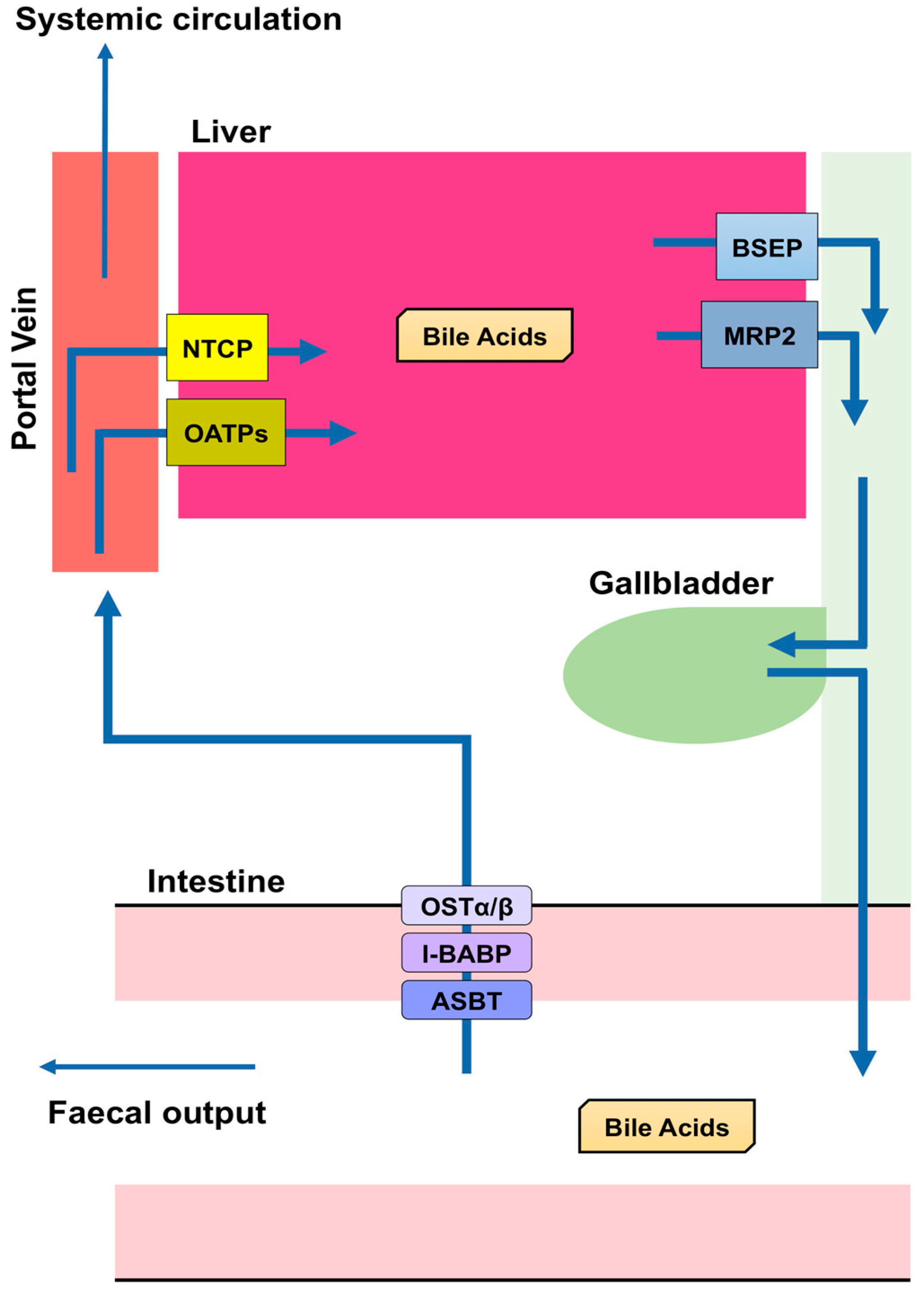
Figure 2. Enterohepatic circulation. Bile salt export pump (BSEP) and multidrug resistance-associated protein 2 (MRP2) are transporters mediating the secretion of bile acids (BA) from hepatocytes to the bile canaliculus in the liver. BA are stored in the gallbladder and secreted into the small intestine after a meal. Most BA (approximately 95%) are reabsorbed, whereas the remainder is excreted with feces. Apical sodium dependent bile acid transporter (ASBT) in the apical brush border of enterocytes takes BA into enterocytes. Ileal bile acid-binding protein (I-BABP) is related to the intracellular transport in enterocytes. The passage of BA through the basolateral membrane of enterocytes into the portal blood occurs through organic solute transporter (OST) α and β. BA released into the blood from the small intestine are transported into the liver by Na+-taurocholate co-transporting polypeptide (NTCP) or organic anion-transporting polypeptides (OATPs). These BA are then reconjugated and secreted with newly produced BA. This recycling system is termed enterohepatic circulation.
After primary BA are transported to and stored in the gallbladder, they are secreted into the small intestine, where the intestinal microbiota converts them into secondary BA. Conjugated primary BA are deconjugated by bacterial bile salt hydrolase [
15,
16]. In humans, 7α-dehydroxylase converts CA and CDCA to deoxycholic acid (DCA) and lithocholic acid (LCA), respectively [
1,
16]. CDCA is also converted to ursodeoxycholic acid (UDCA) by 7α-hydroxysteroid dehydrogenase (7α-HSDH) and 7β-HSDH [
17]. In rodents, β-MCA is transformed into ω-MCA, which is then metabolized to hyodeoxycholic acid (HDCA), while α-MCA is converted to murideoxycholic acid [
18,
19].
Most BA molecules (approximately 95%) are reabsorbed, and the remainders are excreted in the feces [
20]. This reabsorption largely takes place in the small intestine, but a small proportion also occurs in the colon. Apical sodium dependent bile acid transporter (ASBT) in the apical brush border of enterocytes transports BA into enterocytes [
21,
22,
23,
24,
25], and a mutation in this transporter causes primary BA malabsorption [
22]. Although diabetes is a multifactorial disease, appropriate glycemic control is crucial for the prevention of diabetic complications [
26,
27,
28,
29]. Inhibition of the reabsorption of BA by an ASBT inhibitor (GSK2330672) or a BA sequestrant (colesevelam or colestimide) causes a reduction in circulating BA concentrations, which leads to improvements in glycemic control and insulin sensitivity in diabetic patients [
30,
31]. Ileal bile acid-binding protein (I-BABP), also known as fatty acid binding protein 6, mediates intracellular transport in the enterocyte [
21,
32], and the transport of BA through the basolateral membrane of enterocytes into the portal circulation is performed by organic solute transporter (OST) α and β. OSTα and OSTβ are localized to the basolateral membranes of the enterocyte [
21] and expressed as heterodimers or heteromultimers [
33,
34,
35]. BA released into the blood from the small intestine are transported to the liver, reconjugated, and secreted with newly synthesized BA. Thus, BA secreted from the liver into the intestine are recycled, and this recycling system is referred to as the “enterohepatic circulation” ().
The transport of BA from the portal circulation into hepatocytes is performed by Na
+-dependent and Na
+-independent pathways. Na
+-dependent transport of BA requires Na
+-taurocholate co-transporting polypeptide (NTCP), while Na
+-independent transport is executed by organic anion-transporting polypeptides (OATPs). NTCP is localized to the basolateral membrane of hepatocytes and is the main transporter responsible for transporting BA into hepatocytes using a Na
+-dependent mechanism [
36,
37,
38]. OATP1B1 and OATP1B3 are members of the OATP family that are expressed in the basolateral membrane of hepatocytes [
39]. Oatp1b2 is the rodent ortholog of human OATP1B1/3 [
40]. BA conjugated with glycine or taurine are more effectively transported into cells by OATP1B1/3 than unconjugated BA [
41]. However, BA in the portal vein are not completely reabsorbed by hepatocytes (): The concentration of BA in the portal vein is 10–80 μM and that in the systemic circulation of humans and rodents is ∼2–10 μM [
10].
BA in the Brain
Both conjugated and unconjugated BA can be detected in the brain of humans and rodents [
5,
6,
42]. Twenty BA have been identified in the rat brain, consisting of nine unconjugated BA and eleven conjugated BA [
6]. Most of these 20 BA are also found in the blood of rats [
43]. CDCA is present in the highest concentration in the rat brain [
5]. Although the origin of brain BA remains unclear, they can be synthesized in the brain or transported into the brain from the peripheral circulation by BA transporters and/or diffuse across the blood-brain barrier (BBB). Conjugated BA require transporters to cross the BBB, because they are large and negatively-charged at physiological pH [
44,
45], and indeed, transporters for both the uptake and efflux of BA, such as NTCP, OATP1, BSEP, and MRP2, have been identified in the choroid plexus and brain capillaries [
46,
47,
48]. In addition, BA in the bloodstream can themselves induce permeability of the BBB. The BBB is formed by brain microvascular endothelial cells that are bound to adjacent cells via tight junctions [
49]. Occludin is one of the membrane proteins that are responsible for tight junction formation in the BBB [
50], and its phosphorylation by BA via ras-related C3 botulinum toxin substrate 1 (RAC1) increases the permeability of the BBB [
51]. In contrast, unconjugated BA, such as CA, CDCA, and DCA, are lipophilic, even though they are ionized [
52]. Therefore, unconjugated BA might be able to cross the BBB by passive diffusion, and indeed, there are strong correlations between the concentrations of unconjugated BA (CA, CDCA, and DCA) in the brain and the serum [
53,
54].
24
S-hydroxycholesterol predominantly exists in the brain of humans and rodents and is generated from cholesterol in the brain by cytochrome P450 46A1 (CYP46A1), also known as cholesterol 24-hydroxylase [
55,
56,
57,
58] (). 24
S-hydroxycholesterol is a precursor of 3β-hydroxy-5-cholenoic acid, which can be converted to CDCA through the intermediates (3β, 7α-dihydroxy-5-cholenoic acid and 7α-hydroxy-3-oxo-4-cholenoic acid) with rat brain extracts, although this CDCA-generating activity is very low [
59]. Moreover, a large amount of (25R)-26-hydoxycholesterol incorporates to brain from circulation, and (25R)-26-hydoxycholesterol can also be converted to 3β-hydroxy-5-cholenoic acid [
60]. Therefore, it is thought that the principal source of BA in the brain is the peripheral circulation.
References
- Thomas, C.; Pellicciari, R.; Pruzanski, M.; Auwerx, J.; Schoonjans, K. Targeting bile-acid signalling for metabolic diseases. Nat. Rev. Drug Discov. 2008, 7, 678–693.
- Hofmann, A.F.; Hagey, L.R.; Krasowski, M.D. Bile salts of vertebrates: Structural variation and possible evolutionary significance. J. Lipid Res. 2010, 51, 226–246.
- Reschly, E.J.; Ai, N.; Ekins, S.; Welsh, W.J.; Hagey, L.R.; Hofmann, A.F.; Krasowski, M.D. Evolution of the bile salt nuclear receptor FXR in vertebrates. J. Lipid Res. 2008, 49, 1577–1587.
- Molinaro, A.; Wahlstrom, A.; Marschall, H.U. Role of Bile Acids in Metabolic Control. Trends Endocrinol. Metab. 2018, 29, 31–41.
- Mano, N.; Goto, T.; Uchida, M.; Nishimura, K.; Ando, M.; Kobayashi, N.; Goto, J. Presence of protein-bound unconjugated bile acids in the cytoplasmic fraction of rat brain. J. Lipid Res. 2004, 45, 295–300.
- Zheng, X.; Chen, T.; Zhao, A.; Wang, X.; Xie, G.; Huang, F.; Liu, J.; Zhao, Q.; Wang, S.; Wang, C.; et al. The Brain Metabolome of Male Rats across the Lifespan. Sci Rep. 2016, 6, 24125.
- Chiang, J.Y. Bile acids: Regulation of synthesis. J. Lipid Res. 2009, 50, 1955–1966.
- Russell, D.W. Fifty years of advances in bile acid synthesis and metabolism. J. Lipid Res. 2009, 50, S120–125.
- Fakheri, R.J.; Javitt, N.B. 27-Hydroxycholesterol, does it exist? On the nomenclature and stereochemistry of 26-hydroxylated sterols. Steroids 2012, 77, 575–577.
- De Aguiar Vallim, T.Q.; Tarling, E.J.; Edwards, P.A. Pleiotropic roles of bile acids in metabolism. Cell Metab.2013, 17, 657–669.
- Bathena, S.P.; Mukherjee, S.; Olivera, M.; Alnouti, Y. The profile of bile acids and their sulfate metabolites in human urine and serum. J. Chromatogr. B Analyt. Technol. Biomed. Life Sci. 2013, 942–943, 53–62.
- Nakashima, T.; Sano, A.; Seto, Y.; Nakajima, T.; Nakagawa, Y.; Okuno, T.; Takino, T.; Hasegawa, T. Unusual trihydroxy bile acids in the urine of healthy humans. Clin. Chim. Acta 1986, 160, 47–53.
- Li, T.; Apte, U. Bile Acid Metabolism and Signaling in Cholestasis, Inflammation, and Cancer. Adv. Pharmacol. 2015, 74, 263–302.
- Trauner, M.; Boyer, J.L. Bile salt transporters: Molecular characterization, function, and regulation. Physiol. Rev. 2003, 83, 633–671.
- Chikai, T.; Nakao, H.; Uchida, K. Deconjugation of bile acids by human intestinal bacteria implanted in germ-free rats. Lipids 1987, 22, 669–671.
- Begley, M.; Gahan, C.G.; Hill, C. The interaction between bacteria and bile. FEMS Microbiol. Rev. 2005, 29, 625–651.
- Lepercq, P.; Gerard, P.; Beguet, F.; Raibaud, P.; Grill, J.P.; Relano, P.; Cayuela, C.; Juste, C. Epimerization of chenodeoxycholic acid to ursodeoxycholic acid by Clostridium baratii isolated from human feces. FEMS Microbiol. Lett. 2004, 235.
- Martin, F.P.; Dumas, M.E.; Wang, Y.; Legido-Quigley, C.; Yap, I.K.; Tang, H.; Zirah, S.; Murphy, G.M.; Cloarec, O.; Lindon, J.C.; et al. A top-down systems biology view of microbiome-mammalian metabolic interactions in a mouse model. Mol. Syst. Biol. 2007, 3, 112.
- Fu, Z.D.; Csanaky, I.L.; Klaassen, C.D. Gender-divergent profile of bile acid homeostasis during aging of mice. PLoS ONE 2012, 7, e32551.
- Chiang, J.Y. Bile acid metabolism and signaling. Compr. Physiol. 2013, 3, 1191–1212.
- Dawson, P.A.; Karpen, S.J. Intestinal transport and metabolism of bile acids. J. Lipid Res. 2015, 56, 1085–1099.
- Oelkers, P.; Kirby, L.C.; Heubi, J.E.; Dawson, P.A. Primary bile acid malabsorption caused by mutations in the ileal sodium-dependent bile acid transporter gene (SLC10A2). J. Clin. Invest. 1997, 99, 1880–1887.
- Meier, Y.; Eloranta, J.J.; Darimont, J.; Ismair, M.G.; Hiller, C.; Fried, M.; Kullak-Ublick, G.A.; Vavricka, S.R. Regional distribution of solute carrier mRNA expression along the human intestinal tract. Drug Metab. Dispos. 2007, 35, 590–594.
- Shneider, B.L.; Dawson, P.A.; Christie, D.M.; Hardikar, W.; Wong, M.H.; Suchy, F.J. Cloning and molecular characterization of the ontogeny of a rat ileal sodium-dependent bile acid transporter. J. Clin. Invest. 1995, 95, 745–754.
- Christie, D.M.; Dawson, P.A.; Thevananther, S.; Shneider, B.L. Comparative analysis of the ontogeny of a sodium-dependent bile acid transporter in rat kidney and ileum. Am. J. Physiol. 1996, 271, G377–G385.
- Kiriyama, Y.; Nochi, H. Role and Cytotoxicity of Amylin and Protection of Pancreatic Islet β-Cells from Amylin Cytotoxicity. Cells 2018, 7, 95.
- Wali, J.A.; Masters, S.L.; Thomas, H.E. Linking metabolic abnormalities to apoptotic pathways in Beta cells in type 2 diabetes. Cells 2013, 2, 266–283.
- Tuomi, T.; Santoro, N.; Caprio, S.; Cai, M.; Weng, J.; Groop, L. The many faces of diabetes: A disease with increasing heterogeneity. Lancet 2014, 383, 1084–1094.
- Kahn, S.E.; Cooper, M.E.; Del Prato, S. Pathophysiology and treatment of type 2 diabetes: Perspectives on the past, present, and future. Lancet 2014, 383, 1068–1083.
- Hansen, M.; Sonne, D.P.; Mikkelsen, K.H.; Gluud, L.L.; Vilsboll, T.; Knop, F.K. Bile acid sequestrants for glycemic control in patients with type 2 diabetes: A systematic review with meta-analysis of randomized controlled trials. J. Diabetes Complicat. 2017, 31, 918–927.
- Wu, Y.; Aquino, C.J.; Cowan, D.J.; Anderson, D.L.; Ambroso, J.L.; Bishop, M.J.; Boros, E.E.; Chen, L.; Cunningham, A.; Dobbins, R.L.; et al. Discovery of a highly potent, nonabsorbable apical sodium-dependent bile acid transporter inhibitor (GSK2330672) for treatment of type 2 diabetes. J. Med. Chem 2013, 56, 5094–5114.
- Lin, M.C.; Kramer, W.; Wilson, F.A. Identification of cytosolic and microsomal bile acid-binding proteins in rat ileal enterocytes. J. Biol. Chem. 1990, 265, 14986–14995.
- Christian, W.V.; Li, N.; Hinkle, P.M.; Ballatori, N. β-Subunit of the Ostalpha-Ostbeta organic solute transporter is required not only for heterodimerization and trafficking but also for function. J. Biol. Chem. 2012, 287, 21233–21243.
- Ballatori, N.; Christian, W.V.; Lee, J.Y.; Dawson, P.A.; Soroka, C.J.; Boyer, J.L.; Madejczyk, M.S.; Li, N. OSTalpha-OSTbeta: A major basolateral bile acid and steroid transporter in human intestinal, renal, and biliary epithelia. Hepatology 2005, 42, 1270–1279.
- Dawson, P.A.; Hubbert, M.; Haywood, J.; Craddock, A.L.; Zerangue, N.; Christian, W.V.; Ballatori, N. The heteromeric organic solute transporter alpha-beta, Ostalpha-Ostbeta, is an ileal basolateral bile acid transporter. J. Biol. Chem. 2005, 280, 6960–6968.
- Ananthanarayanan, M.; Ng, O.C.; Boyer, J.L.; Suchy, F.J. Characterization of cloned rat liver Na(+)-bile acid cotransporter using peptide and fusion protein antibodies. Am. J. Physiol 1994, 267, G637–G643.
- Stieger, B.; Hagenbuch, B.; Landmann, L.; Hochli, M.; Schroeder, A.; Meier, P.J. In situ localization of the hepatocytic Na+/Taurocholate cotransporting polypeptide in rat liver. Gastroenterology 1994, 107, 1781–1787.
- Hagenbuch, B.; Scharschmidt, B.F.; Meier, P.J. Effect of antisense oligonucleotides on the expression of hepatocellular bile acid and organic anion uptake systems in Xenopus laevis oocytes. Biochem J. 1996, 316, 901–904.
- Nigam, S.K.; Bush, K.T.; Martovetsky, G.; Ahn, S.Y.; Liu, H.C.; Richard, E.; Bhatnagar, V.; Wu, W. The organic anion transporter (OAT) family: A systems biology perspective. Physiol. Rev. 2015, 95, 83–123.
- Csanaky, I.L.; Lu, H.; Zhang, Y.; Ogura, K.; Choudhuri, S.; Klaassen, C.D. Organic anion-transporting polypeptide 1b2 (Oatp1b2) is important for the hepatic uptake of unconjugated bile acids: Studies in Oatp1b2-null mice. Hepatology 2011, 53, 272–281.