1. Origin of Metal Matrix Composites
Composite materials are defined as those hybrid materials consisting of two or more materials, with clearly distinct interfaces between the constituents. More specifically, the term composite is restricted to those materials that are composed of a continuous matrix, which binds together the discrete phases corresponding to the reinforcement constituent. Different types of reinforcements can be employed to constitute composite materials, namely discrete and continuous. The final properties of the composite materials differ substantially from the properties of their constituents. Indeed, the final properties of the composite materials are derived from the individual attributes of the constituents. Metal matrix composites (MMC) refer to those composites constituted by a metallic matrix and typically reinforced by a ceramic phase
[1]. In MMC material systems, the metallic phase serves as a binder to the composite, while the ceramic phase acts as the reinforcement. MMCs emerged in the 1960s to further improve the mechanical properties of structural superalloys for applications related to defence and aerospace
[2][3]. Later, in 1991, the suitability of these materials for the surface modification of components exposed to critical erosion and wear conditions was established
[4]. At that time, the synthesis of MMCs was barely possible, which is why not as many extensive and comprehensive studies have been carried out in this field. However, owing to the rapid development of AM and the recent popularity of multi-material AM, the research interest in MMCs has risen accordingly.
In terms of the industrial requirements, the development of MMCs has been driven by the need for complex materials with a higher hardness to produce wear-resistant coatings for high-end applications
[5][6]. This problem has been traditionally addressed through alloy design, i.e., by developing adequate alloys to meet the requirements set by the industry. In this regard, hardfacing with Fe-, Ni-, and Co-based alloys using L-DED for wear- and corrosion-resistant coating applications have been broadly investigated
[7][8][9]. Furthermore, alloying with elements such as niobium, vanadium, and tungsten has been demonstrated to extend the tooling lifetime
[10]. However, every so often, monolithic materials fail to provide a suitable solution for industrial problems
[11], and when an additional improvement of the wear resistance of metal alloys is required without compromising their toughness, composite materials are typically considered. In fact, in monolithic alloys, increasing the hardness of materials typically comes at the expense of a loss in ductility
[12]. Conversely, ceramic-reinforced MMCs yield superior material properties provided by the individual contributions of their constituents, e.g., the high hardness and high strength-to-weight ratio of the ceramic phase, in combination with the high toughness and ductility of the metal matrix
[13]. Therefore, surface engineers often resort to ceramic hard metal coatings to increase the resistance to wear
[14]. Fortunately, laser processing technologies enable a localised dispersion of ceramic particles
[15] or the deposition of ceramic particle-reinforced MMC coatings
[16].
2. Advantages and Applications of Metal Matrix Composites
MMCs benefit from the high hardness, strength, and wear resistance of the ceramic phase, but also from the high ductility and good electric and thermal properties of the metallic phase
[17]. Overall, ceramic-reinforced MMCs have been proven to offer superior properties in terms of their strength, hardness, wear, and corrosion resistance, and also to behave well even when exposed to high-temperature conditions
[18]. The reinforcement phase can strengthen the metal binder in many ways, with coating applications being in the spotlight
[19]. On the one hand, when the composite material is subjected to an external load, it is transferred from the metal matrix to the ceramic particles in an efficient manner
[20]. On the other hand, the discrete particles inhibit the movement of dislocations and restrict plastic deformation. As a consequence, the metallic phase is retained and the wearing out is prevented
[18]. This is the reason behind the higher wear resistance of MMCs over monolithic alloys
[21].
Owing to the outstanding behaviour of MMCs, they have been widely proposed to improve the surface properties of highly demanded components, as an alternative to conventional metallic alloy systems
[22]. They are of particular interest in those applications where conventional alloys lack sufficient hardness and wear resistance
[23], such as in aerospace
[24], die and mould
[25], agricultural
[26][27], mining
[28], automotive
[29], and oil and gas applications
[30]. In short, they are an interesting solution for those applications where wear, erosion, and corrosion are the main mechanisms responsible for the failure of components
[31].
3. Production of Metal Matrix Composites
Back when the potential of MMCs was barely glimpsed, their production was strongly limited by the processing techniques available at the time, as their manufacture by conventional methods is complicated
[18]. The low ductility and low fracture toughness are extremely challenging and limit their processability using casting or powder metallurgy techniques
[32]. In the last decade, and owing to the rise of AM technologies, namely L-DED, a significant effort has been invested into researching this topic. According to Bandyopadhyay et al., if the challenges related to the production of MMCs are overcome, high-performance components with good behaviour could certainly be attained
[19]. Despite the production of these material systems remaining problematic, according to Mostafei et al., L-DED is a reliable method for manufacturing MMCs, and it is particularly suitable for the deposition of MMC coatings
[33]. As a matter of fact, the high flexibility in terms of the materials and the tight control of the composition of the multi-material mixtures place L-DED in a highly competitive position for the production of MMC parts and coatings
[5][34].
MMC structures are typically fabricated through powder-based processes, where the ceramic and the metallic powders are premixed in ratios ranging from 1 to 20 wt. %
[35]. Nonetheless, mixtures containing higher amounts of the ceramic phase are also frequently reported. The L-DED of MMCs can be approached in two different ways, depending on the mechanism for the formation of the reinforcement phase
[32], see
Figure 1.
Figure 1. Formation mechanisms of MMCs by L-DED. Images reproduced from [36][37].
-
Ex situ production of MMC (Figure 1a): The first approach consists of the projection of a powder mixture with a precise volumetric fraction of the ceramic phase into the melt pool. In either case, the reaction between the ceramic and the metallic phase is limited and controlled. To this end, the process parameters and the feedstock morphology and granulometry should be selected so as to guarantee that no excessive dissolution of the ceramic phase occurs. In addition, the process parameters should be selected so as to guarantee proper bonding between phases.
-
In situ production of MMC (Figure 1b): In the second approach, a mixture of elemental powders is introduced into the melt pool. The high processing temperatures used in L-DED allow chemical reactions between elements to occur, resulting in the formation of disperse carbides or intermetallics. Conversely, a ceramic–metallic powder mixture can be fed but the complete decomposition of the ceramic phase must be ensured so that the in situ synthesis of dispersed carbides takes place. In this manner, MMCs may be in-situ-synthesised. In both cases, the process parameters and the powder morphology should be carefully selected to facilitate the in-situ synthesis of carbides.
According to the formal definition of the composites, the material systems obtained through the second approach do not correspond to MMCs but rather to alloys with dispersed hard phases, as no macroscopic phases could be distinguished. However, these complex alloys are often referred to as MMCs in the literature. In addition, controlling the dissolution of the ceramic phase in ex-situ-produced MMCs is not a trivial matter. In ceramic-reinforced MMCs produced via L-DED, the ceramic particles often suffer partial dissolution, which promotes interfacial reactions between the ceramic and metallic materials
[38], as showin in
Figure 2. As a result, complex hierarchical structures are often observed. This phenomenon has been investigated in the literature; hence, the factors governing it and its consequences are more extensively discussed in the next section.
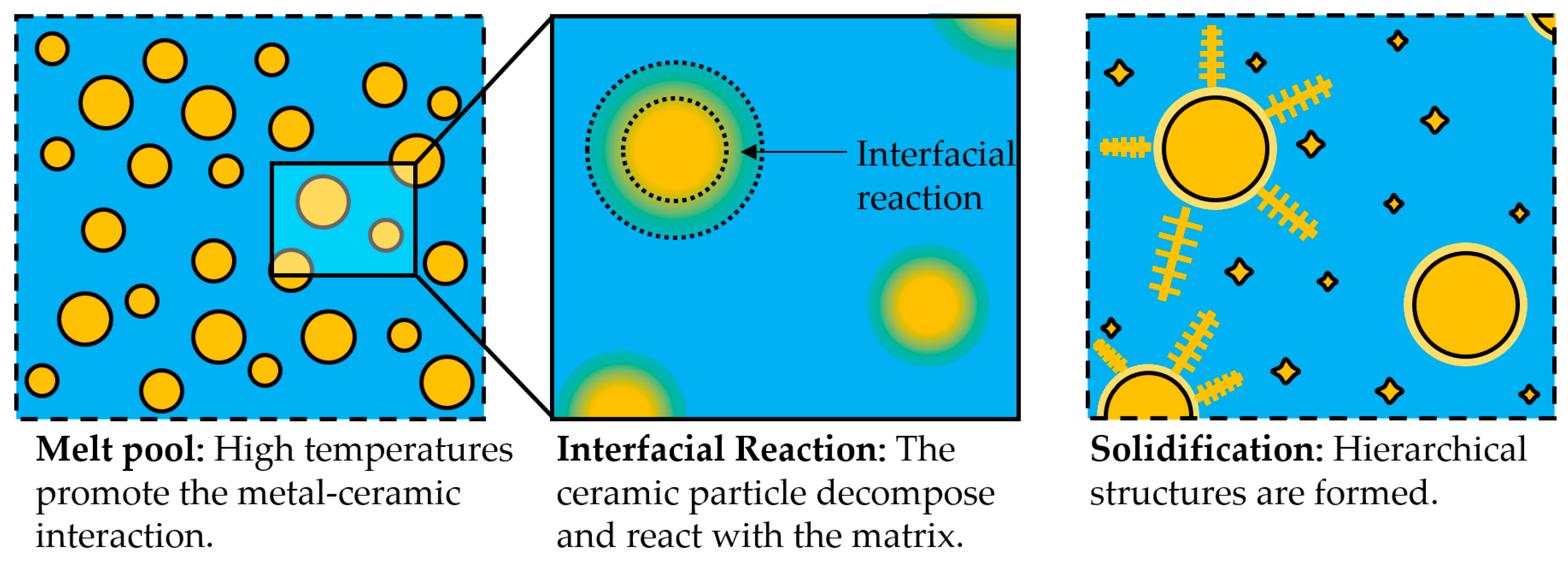
Figure 2. Schematic illustration of the metal-ceramic interaction during L-DED of MMC coatings and formation of hierarchical structures.
In essence, L-DED is a highly competitive technology that has the potential to form MMC structures, owing to the high flexibility when processing the multi-material structures
[39]. At the same time, L-DED enables the deposition of high-quality surface coatings, which is the main application of ceramic-reinforced MMCs. Driven by this motivation, several aspects of the production of MMCs through L-DED have been investigated. Accordingly, the most relevant studies in the literature are summarised.
4. Most Relevant Literature on L-DED of Metal Matrix Composites
The research carried out in this field has focused on the analysis of different material systems and their performance. For instance, Nurminen et al. compared various material systems consisting of a metal binder and disperse carbides
[40]. They focused on addressing material compatibility issues and their effect on the performance from a tribological perspective. They concluded that the properties of MMCs were strongly linked to the material selection and the chemical affinity between the constituents. Indeed, material systems with high affinity would promote the decomposition of the ceramic phase, which eventually was found to be detrimental to the performance of the coating. Jiang and Kovacevic fabricated MMC coatings containing TiC and AISI H13 tool steel and compared the tribological behaviour of this material system to other material systems previously reported in the literature
[38]. Adam et al. investigated the performances of different material systems for ballistic applications and studied the suitability of L-DED for the production of MMC coatings
[41]. Zhang and Kovacevic investigated the tribological performance of MMC coatings composed of an AISI 420 steel matrix and different carbides with the aim of providing some insights on material selection
[30]. These studies demonstrated the adequacy of MMC coatings manufactured by means of L-DED for the surface modification of components exposed to severe operating conditions and outlined some guidelines to facilitate material selection. However, a limited focus was placed on understanding how the processing conditions affect the performance of MMC coatings within a sole material system. Hence, generic conclusions may be drawn, but no deep understanding was gained concerning the specific microstructures that should be targeted and no methodologies were proposed to materialise it.
Other studies focused on the microstructural evolution of MMC coatings due to the high-temperature processing of L-DED. As mentioned above, the dissolution of the ceramic particles in ex-situ-produced MMCs is hardly avoidable, and this phenomenon affects both the microstructural aspects and mechanical properties. Li et al. investigated (Cr, W)
23C
6- and WC-reinforced Fe-based composite coatings. They reported the phase transformations occurring as a result of the L-DED process. Disperse eutectic carbides were found in the matrix structure, but fine carbides also precipitated in the interdendritic phase
[23]. Along the same line, Zhao et al. investigated the dissolution of the reinforcement phase for WC-Ni material systems in
[42] and WC-Fe in
[43]. In their studies, a thorough characterisation process of the microstructure generated as a result of the interaction between the reinforcement phase and the matrix phase was carried out. They observed that carbides with different morphologies were dispersed in the metal matrix, while retained particles were also encountered. In addition, the main mechanisms responsible for the decomposition of the ceramic particles were reported, namely dissolution, diffusion, partial or complete fragmentation, and precipitation. From a processing perspective, Muvvala et al. investigated the metal–ceramic interface in a WC-reinforced Ni-based alloy. They found that the decomposition of the reinforcement particles played a major role in the bonding type. Moreover, they measured the melt pool lifetime and correlated it with the thickness of the reaction layer surrounding the ceramic particles
[44].
These studies have brought to light the importance of the interactions between the constituent phases in MMC composites, especially considering the high temperatures inherent to L-DED processing. However, few studies have been carried out involving a thorough investigation and discussion of the effects of the process parameters and the thermal cycle of the manufacturing process. In fact, none of the previous authors found a correlation between the process parameters, microstructure, and resulting properties of the coatings. Hence, the role of the processing parameters in the metal–ceramic interactions and the microstructures and properties of MMC coatings has been neglected, especially in the formation of unexpected microstructural phases. It is out of the question that the L-DED parameters are responsible for the thermal cycle generated during processing. Thus, the process parameters affect the dissolution of the reinforcement particles, and presumably the mechanical properties, such as the hardness, of MMC coatings.
In this line, another aspect that has attracted the attention of researchers is the hardness of MMC coatings and the correlation between the hardness and the volumetric fraction of the reinforcement phase. Most authors report that increasing the volumetric fraction of the reinforcement phase results in a substantial enhancement of the hardness. Li et al. observed a gradual increase in hardness with increasing volumetric fractions of WC from 0% to 20%. They ascribed this to the formation of carbides and the solid–solution strengthening due to lattice distortion
[23]. Xie et al. and Raahgini et al. reported a nearly linear correlation between the hardness and the volumetric fraction of the reinforcement phase for Co-WC
[45] and Ni-VC
[21] material systems, respectively. Deschuytenner et al., on the other hand, characterised the multi-scale hardness of a WC-reinforced Ni-based alloy. In short, they studied the normalised hardness of the composite samples, but also the hardness achieved by the metal matrix as a result of the microstructural modification
[46]. Ostolaza et al. investigated the effect of the processing parameters and the feedstock composition on the hardness of the matrix and on the hardness of the composite in WC-Co coatings through statistical regression methods
[47]. It was concluded that longer interaction times promoted the decomposition of the ceramic particles, which resulted in an increase in the W content in the matrix. At the same time, different mechanisms responsible for the hardening of the MMC coatings were reported, namely grain refinement and carbide precipitation, depending on the feedstock composition and processing conditions. Zhao et al., on the other hand, quantified the loss in hardness when the dilution of the substrate increased
[42]. As expected, the higher the amount of substrate material that was melted, the lower the hardness of the coating material. Ultimately, having higher substrate dilution alters the composition of the coating. If the substrate material is softer than the coating material, then the overall hardness of the coating will decrease.
Lastly, many authors have tried to demonstrate the suitability of MMCs for high-performance coating production by studying their wear resistance. Li et al. investigated the tribological behaviour of (Cr, W)
23C
6-WC-reinforced, Fe-based coatings. They obtained good results in terms of the wear resistance of the MMC samples. More interestingly, they found that the surface properties were maximised for an optimal coating composition
[23]. Xie et al. studied the wear resistance of WC-Co MMCs and reported a positive correlation between the wear resistance and the WC content of the MMC
[45]. Similar conclusions were reached by Zhao et al. for WC-Fe
[43] and WC-Ni
[42] MMC coatings. Their experimental results suggested that a significant gain in tribological performance could be obtained by increasing the WC content. They ascribed this effect to two phenomena, namely the retained WC particles that remained unmelted and the dispersed carbides throughout the matrix. Bartkowski and Kinal achieved increased wear resistance by embedding WC particles into a Stellite 6 matrix as compared to the monolithic Stellite 6 coatings
[26]. They concluded that the excess WC content or insufficient hardness of the matrix could promote a more intensive wear mechanism in MMC coatings. In a later publication, they obtained promising results when testing the behaviour of the designed coating in real conditions when applied to an agricultural tool. They reported a 25% increase in the life of the tooling
[27].
In this regard, Muvvala et al. carried out a comprehensive investigation on the contribution of the ceramic phase to wear resistance
[28]. They concluded that the uncontrolled dissolution of the ceramic particles caused the embrittlement of the matrix phase. As a result, the fracturing and spalling of the surface during wear testing were promoted; hence, these samples suffered a higher wear rate. It can be concluded that increasing the reinforcement content does contribute to a higher wear resistance of MMC coatings, provided that the ceramic particles do not fracture and that the third body mechanism is avoided
[21]. Similar conclusions were previously reached by Fernández et al.
[48]. In MMC coatings, the discrete reinforcement particles rule the plastic flow of the metal matrix. Hence, increasing the reinforcement phase changes the main wear mechanism; indeed, it switches from severely adhesive to mildly adhesive and abrasive wear
[49].
The high-temperature wear resistance of Co- and Ni-based MMC coatings has also been investigated. Erfanmanesh et al. studied the high-temperature tribological behaviour of WC-reinforced Ni- and Co-based matrices
[50]. The reinforced samples showed superior wear resistance and they found that soft abrasive and adhesive mechanisms were responsible for the wear damage. Wang et al. also tested the high-temperature wear resistance of WC-Co MMC coatings and confirmed the previously reported good behaviour of this material system
[25]. In addition, the experimental results showed a positive correlation between the wear resistance with respect to the WC content. However, they reported a significant drop in the thermal fatigue life when increasing the WC content over 20% wt. Lastly, Karmakar et al. investigated the abrasive wear of WC-Co- and Co-coated AISI H13 tool steel substrates at high temperatures
[8]. The ceramic-reinforced coatings yielded greater resistance to wear up to 650 °C as compared to the un-coated substrates. The superior surface properties of the reinforced coatings were more evident the higher the temperature of the abrasive test.
Although the behaviour of MMC coatings in terms of wear has been thoroughly studied, other aspects that tightly limit their performance have been completely neglected. For instance, little effort has been devoted to the evaluation of the interfacial strength between the MMC coating and the substrate, which will directly affect the durability of the surface-treated components. Indeed, the flexural strength of coated parts and the residual stresses induced during processing are key aspects that limit the life and the safe operation of coated components
[51]. It is without question that the surface properties and mechanical behaviour of MMC coatings are ruled by the strength of the interfacial bonds between the macro-constituents, as has been stated by several researchers
[52][53]. On the one hand, the reaction layer guarantees a metallurgical bond; therefore, the cohesion of the composite is ensured and one should expect proper load transfer between the macroscopic constituents
[53]. Conversely, the dispersion of discrete carbides throughout the matrix may cause a loss in ductility, which is detrimental to the structural behaviour of the composites
[54]. Thus, the key lies in finding a balance in the extent of the metal–ceramic interaction. Metallurgical bonding between the matrix and the reinforcement should be sought, but the embrittlement of the metal matrix should be prevented to preserve the good mechanical properties of the matrix. This issue may be solved by optimising the process parameters and by tuning the thermal cycle during processing
[28].
In terms of the processability of MMCs through L-DED, the realisation of good-quality coatings is still a challenge. For instance, material incompatibility is an issue that needs to be tackled
[55]. Moreover, defects related to the metallurgical integrity (pores or cracking) are frequently encountered
[33].
The cracking of MMCs deposited by L-DED has been widely studied in the literature
[18]. Cracks in these coatings originate from the high residual stresses generated during the deposition process
[33]. As a process based on the fusion and rapid solidification of materials, parts manufactured by L-DED withstand high temperature gradients; thus, high thermal stresses can be produced if specific care is not taken
[18]. In addition, the interaction between the particles and the matrix tends to provoke matrix embrittlement. The loss of ductility prevents the metal matrix from absorbing the residual stresses of the manufacturing process. Additionally, the reinforcement particles act as stress concentrators
[8], which further exacerbate cracking. Consequently, it has been stated in the literature that these materials often suffer not only from cracking phenomena but also other sorts of catastrophic failures due to high residual stresses and material property mismatches, such as delamination
[56]. Multiple methods have been proposed to eliminate cracking, namely substrate preheating, the addition of rare earth oxides, or the elimination of the sharp transition between substrates and coatings through the use of functionally graded materials (FGMs)
[16].
5. Conclusions
The ability of L-DED to manufacture multi-material components is one of the most compelling aspects of this technology, and so it has been acknowledged by industry and research bodies. The current challenges related to joining dissimilar materials, for instance, can be overcome through the use of multi-material L-DED, as components composed of different materials can be built up in a single operation. The prevalent applications of multi-material L-DED are alloy design, metal matrix composites, and functionally graded materials. Surface engineers can obtain major benefits from metal matrix composite coatings, as they behave outstandingly well in terms of wear.
The straightforward ability of L-DED to control the feedstock composition and modify it as an additional process parameter has provided evidence of the suitability of this process for multi-material fabrication, and it is already considered the prevailing technology for forming multi-material structures. However, some issues need to be addressed before this technology is ready for industrial use. Some of these challenges concern process engineering and materials science, which are summarised below.
In terms of process engineering, the composition of the feedstock injected into the melt pool needs to be tightly controlled. If the L-DED system involves several powder hoppers, then the individual mass flow rate of each one of the hoppers needs to be precisely controlled, and the dynamic behaviour when varying the composition of the feedstock during the build-up should be carefully understood. Downstream, the fluid dynamic behaviour of multi-material powder mixtures needs to be known. Indeed, the concentration of the powder mixture by the nozzle depends on the material properties. This is especially troubling when working with metal–ceramic powder mixtures, as the inertial properties of ceramic and metallic materials differ significantly. Failure to understand and predict the behaviour of the powder feeding system from the powder feeder to the injection of the powder mixture into the melt pool will inevitably cause deviations between the nominal composition and the real one.
Moreover, in view of the impact of the thermal cycle during processing on the properties and integrity of multi-material L-DED, the effect of the process parameters on the thermal history should be investigated. Eventually, the process parameters will be tuned to target a specific thermal cycle. Moreover, in the case of MMCs, the thermal history will be defined and controlled to find a balance in the interactions between the ceramic and the metallic phases. With regard to materials engineering, a deeper understanding should be gained of how the thermal cycle affects the interactions between the constituents in a multi-material powder mixture. Considering that the non-equilibrium synthesis prevails in L‑DED, extensive databases should be developed to ease the set-up process and target or avoid specific microstructures. In addition, the in-service behaviour of multi-material structures needs to be tested, whether it be the tribological performance or the structural behaviour. Based on that knowledge, generic guidelines should be drawn so that the material selection and the choice of processing conditions are facilitated.
This entry is adapted from the peer-reviewed paper 10.3390/ma16041746