Despite the new insights that have been brought into the role of redox mechanisms in plant defense response, still one of the major challenges is to monitor local, subcellular, and global ROS dynamics with high selectivity, sensitivity, and spatiotemporal resolution that allow for quantification
[82]. Small organic-molecule-based probes have been originally used to measure ROS in plants; however, they have significant limitation as they do not allow for spatiotemporal resolution, since the fluorescence changes as a result of ROS presence are irreversible
[83][84]. Nondestructive real-time measuring of redox state in plants with high spatial and temporal resolution is feasible since Jiang et al. reported the use of redox-sensitive green fluorescent protein (roGFP)
[85] (
Figure 2). Measurement of roGFP fluorescence intensity following excitation with two different wavelengths enables the calculation of the ratio between reduced and oxidized roGFP and thus the determination of the redox state on the cellular or organelle level. The biosensors have been modified by the addition of signal sequences to target them to different subcellular organelles, while two variants, roGFP1 and roGFP2, with different excitation and emission spectra, were developed to allow for optimal selection according to the redox state in the particular organelle. By fusing the peroxisomal targeting peptide sequence SKL, per-roGFP1 and px-roGFP2 were targeted to peroxisomes
[86][87]. mt-roGFP1 and mt-roGFP2 are available for measuring the redox state in mitochondria due to the fusion with mitochondrial localization signal peptide from the tobacco b-ATPase
[85][87], while er-roGFP2 was constructed by fusing roGFP2 with the endoplasmic reticulum (ER) retention signal peptide HDEL for following the redox state in ER
[88]. Finally, cp-GFP2, pt-roGFP2, and chl-roGFP2 were constructed by adding plastid-targeting signal peptide TKTP, coding sequence for RuBisCo small subunit transit peptide, or the first 74 amino acids from PRXa to roGFP2 coding sequence, respectively, for measuring change of redox state in chloroplasts
[71][89][90]. Since pt-roGFP is targeted to the chloroplast outer plastid envelope membrane, it also allows for the imaging of stromules formation
[68]. The above-mentioned sensors were used in tobacco, Arabidopsis, and potato subjected to different developmental and environmental stresses
[68][71][85][86][87][88][89][90][91][92][93][94], some of them also in HR
[58][95][96]. roGFP2 expressed in the cytosol senses the redox potential of the cellular glutathione buffer via glutaredoxin (GRX) as a mediator of reversible electron flow between glutathione and roGFP2
[88]. To facilitate specific real-time equilibration between roGFP2 and the glutathione redox couple, fusion constructs with human glutaredoxin 1 (GRX1) Grx1-roGFP2 and roGFP2-Grx2 were generated
[97][98][99]. Moreover, two roGFP derivatives, roGFP2-iL and roGFP1-iX, with different midpoint potential and excitation properties, were developed to further extend the range of suitable probes
[100]. Another group of genetically encoded sensors that detect H
2O
2 levels instead of measuring glutathione redox potential was also developed. roGFP2-Orp1, based on a redox relay between the GPX-like enzyme oxidant receptor peroxidase-1 (Orp1) from
Saccharomyces cerevisiae and roGFP2 was generated for sensing transient changes in H
2O
2 [93]. Another sensor that reports on local alterations in H
2O
2 concentrations exploits the H
2O
2-sensitive bacterial transcription factor OxyR for its response and was named HyPer
[101][102]. The above-mentioned types of sensors were also used to follow redox potential and H
2O
2 in the cytoplasm during HR
[103][104][105][106][107][108] or were further upgraded to follow redox potential in different cellular compartments, including chloroplasts
[109]. Using HyPer2, Exposito-Rodriguez et al. showed that in photosynthetic
Nicotiana benthamiana epidermal cells, exposure to high light increased H
2O
2 production in chloroplast stroma, cytosol, and nuclei, suggesting direct H
2O
2 transfer from chloroplasts to nuclei
[110]. Other genetically encoded redox and H
2O
2 sensors are summarized in
[82][111]. The most recent ones are biosensor CROST for measurements of the thioredoxin redox state in chloroplasts
[112], FRET-based biosensors, and ROS regulated promoter–FP fusions
[82][113].
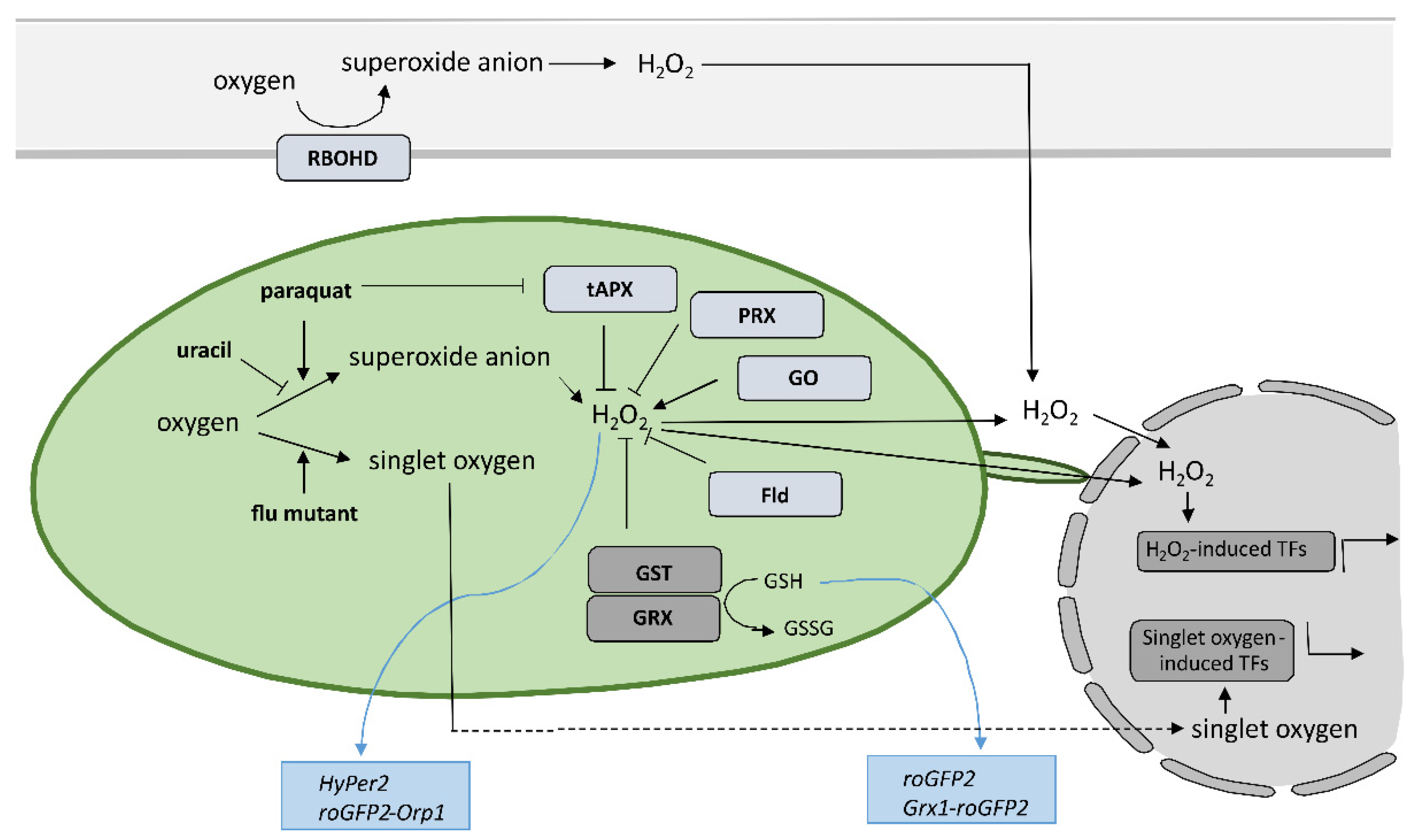
Figure 2. Tools to investigate the role of specific reactive oxygen species produced in chloroplasts and apoplasts.
Another strategy for studying the role of organelle-specific redox state is by altering ROS production in the organelle of interest. Chloroplasts produce various forms of ROS during photosynthesis. One of the most reactive ones is singlet oxygen, which is produced by energy transfer from excited triplet state chlorophyll to the oxygen, mainly in the photosystem II reaction center
[114]. The other major source of chloroplastic ROS is Mehler reaction in photosystem I, which reduces oxygen to superoxide anion that is further converted to H
2O
2 by thylakoid-bound and stromal superoxide dismutases
[115]. H
2O
2 is reduced in reactions catalyzed by 2-Cys peroxiredoxin (PRX) and ascorbate peroxidase (APX)
[116]. Therefore, the role of chloroplastic ROS could be studied in transgenic plants with overexpressed
thylakoidal ascorbate peroxidase (
tAPX), which results in decreased chloroplastic ROS production
[56][117] or in transgenic plants with estrogen-inducible RNAi silenced
tAPX expression, which results in increased chloroplastic ROS production
[61]. Inducible silencing of
tAPX increased H
2O
2 production in chloroplasts, which activated SA biosynthesis and SA-inducible gene expression
[61]. Interestingly, however, over-expression of
stromal ascorbate peroxidase (
sAPX) or treatment with photosynthesis inhibitor DCMU attenuates nuclear H
2O
2 accumulation and high-light-responsive gene expression, while
cytosolic ascorbate peroxidase overexpression has little effect
[110]. This was explained by the direct H
2O
2 transfer from chloroplasts to nuclei, avoiding the cytosol, which enables photosynthetic control over gene expression
[110]. As PRX similarly as APX reduces H
2O
2 accumulation, silencing of
PRX results in enhanced chloroplastic H
2O
2 accumulation. By VIGS-induced
PRX silencing, Ishiga et al. showed that PRX functions as a negative regulator of pathogen-induced cell death in the healthy tissue that surrounds the lesions, while chloroplastic ROS play a role in the cell death initiation
[53]. The role of chloroplastic ROS could also be studied by the use of transgenic plants with chloroplast-targeted flavodoxin (Fld)
[118]. Fld improves the delivery of reducing equivalents to productive pathways of the chloroplast, which in turn restricts chloroplastic ROS production. The introduction of a Fld in chloroplasts of various plant species resulted in increased tolerance to different biotic and abiotic stresses
[51][118][119][120][121][122][123]. Plants overexpressing
glycolate oxidase (
GO) are another system for studying the effects of chloroplastic H
2O
2 [124]. By exploiting this system, Schmidt et al. showed that H
2O
2 dosage in Arabidopsis chloroplasts regulates HR-conferred resistance to hemibiotrophic fungus by the induction of WRKY33
[125]. While the above-mentioned systems modulate chloroplastic H
2O
2 accumulation, Arabidopsis
flu mutant manifest increased singlet oxygen production in chloroplasts upon dark/light shift
[126], which leads to induced SA synthesis and suppressed spread of necrotic lesions
[57]. ROS production in the chloroplasts can also be enhanced by using inhibitors and redox catalysts. The herbicide methyl viologen (paraquat) acts by re-directing electrons from photosystem I (PSI) to oxygen and thereby enhancing the production of superoxide in the chloroplasts
[127]. As it also inhibits APX, this leads to accumulation of H
2O
2 in treated plants
[128]. To study the role of chloroplast-derived photo-oxidative stress in different cellular components, Ugalde et al. treated Arabidopsis seedlings with methyl viologen and recorded dynamic changes in glutathione redox potential and H
2O
2 levels with the genetically encoded biosensors Grx1-roGFP2 and roGFP2-Orp1 targeted to chloroplasts, the cytosol, or mitochondria
[109]. Similarly, the role of chloroplastic ROS was studied using uracil, a chloroplast electron transport chain inhibitor, which significantly reduced ROS generation and delayed necrosis appearance in biotic stress
[129].
6. Conclusions
The results of the above-mentioned studies suggest that the precise spatiotemporal regulation of key players, including organelle-specific ROS and SA levels, determines the effectiveness of pathogen arrest and is therefore crucial for a successful immune response. The change of SA and ROS levels and other key players alter the rate of cell-to-cell and systemic pathogen spread, rate of cell death induction, and spatial transcriptional response, leading to susceptibility or resistance. Scholars suggest that only a coordinated and intertwined action of all main components enable effective immune response. However, the specific interactions between them and the regulatory interplay behind still remain poorly understood, as up until now, the role of organelle-specific ROS and SA in HR-conferred resistance has only been studied by altering the level of a single component. In order to address these aspects, a sophisticated combination of research methods for monitoring the spatiotemporal dynamics of key players and transcriptional activity in plants is needed. The precise sampling of tissue sections surrounding the HR-PCD, with spatial resolution and suitable for transcriptomics analyses [11], in combination with the use of biosensors [130], could enable identification of novel key players and could unravel the interconnectivity of immune signaling components. Such an approach could therefore present a step forward in studying the resistance response.