1. Food Intake and Gut Microbiota (GM) -Mediated Cardiovascular Disease (CVD) Risk
Depending on its composition, GM can transform nutrients present in the food into protective or damaging products entailing healthful or noxious effects, respectively
[1]. The most representative examples of bioactive GM-derived compounds are: (1) metabolites of cholesterol such as primary bile acids (PBAs), secondary bile acids (SBAs), and coprostanol, (2) trimethylamine (TMA)/trimethylamine N-oxide (TMAO), (3) phenyl-acetyl-glutamine (PAG), (4) short chain fatty acids (SCFAs), and (5) polyphenols
[2]. Some of these molecules have been proved to functionally interact with SH, which may explain at least in part, the gender dimorphism in CV risk and CVD outcomes
[3].
1.1. Bile Acids Modulation and Cholesterol Metabolism
Bile acids (BAs) are considered critical components of the crosstalk between GM and cardiovascular (CV) health. The GM can directly influence the hepatic regulation of cholesterol metabolism
[4] and alter bile acid composition and abundance, which in turn affect systemic cholesterol levels
[5]. Originating from cholesterol catabolism, BAs are synthesized in the liver as primary bile acids (PBAs), then conjugated to amino acids taurine or glycine to form bile salts and included in the bile. Next, the bile is secreted in the duodenum to facilitate digestion and absorption of fats, nutrients, and liposoluble vitamins
[6]. In the intestine, PBAs are deconjugated by bile salt hydrolase (BSH), an enzyme produced by a broad spectrum of aerobic and anaerobic bacteria (Gram-positive
Bifidobacterium,
Lactobacillus,
Clostridium, and
Enterococcus and Gram Negative
Bacteroides). Later, a microbial-mediated dehydroxylation converts PBAs into secondary bile acids (SBAs). This reaction is catalyzed by a limited number of bacteria belonging to different genera (
Bacteroides,
Clostridium,
Eubacterium,
Lactobacillus, and
Escherichia)
[7].
PBAs appear to play a role in preventing the development of atherosclerosis through activation of the farnesoid X receptor (FXR) signaling cascade, which improves lipid profile and regulates gluconeogenesis and intestinal barrier function
[8]. In addition, PBAs inhibit the NF-kB-dependent activation of Takeda-G protein 5 receptors (TGR5), resulting in a decreased production of proinflammatory cytokines
[2][9]. PBAs can also influence CV function and reduce heart rate by regulating channel conductance and calcium dynamics in sinoatrial and ventricular cardiomyocytes. Finally, PBAs modulate vascular tone through a transcriptional regulation of vasoactive molecules as long-lasting effect
[10].
Some studies have demonstrated an association between altered plasma or fecal BAs concentrations and CVD risk
[11]. In particular, high concentrations of SBAs have been related with atherosclerosis development and CVD through GM-mediated mechanisms
[12]. In fact, BSHs of GM are able to hydrolyze glycine and taurine conjugates to liberate free BAs; the resulting SBA overproduction promotes enhanced cholesterol levels and increases foam cell formation and atherosclerotic plaque size
[9]. Accordingly, a low SBA excretion represents an independent risk factor for stroke and mortality
[11]. Moreover, an alteration of SBAs to PBAs ratio may be implicated in hypercholesterolemia and CAD development. In this regard, Meyerhofer et al. demonstrated that higher plasma levels of SBAs in front of reduced PBAs in heart failure (HF) patients are associated with worse outcomes in univariate analysis
[10]. On the other hand, some species of bacteria produce less absorbable SBA molecules that are excreted in the stool. This process stimulates BA neo-synthesis by the liver, leading to a net loss of circulating low-density lipoprotein (LDL) and reduced CV risk
[13].
Collectively, the available findings indicate that GM plays a pivotal role in BAs synthesis and in determining the types and amount of PBAs and SBAs, with beneficial or harmful effects on health depending on the composition of the bacteria population.
Another mechanism involving GM in cholesterol metabolism is the conversion of absorbable cholesterol to coprostanol, a reduced non-absorbable sterol
[14]. Although further studies are needed to better clarify this pathway, it is conceivable that GM may contribute to lower blood cholesterol and CV risk also by enhancing cholesterol removal through this route. The rate of GM-mediated cholesterol-to-coprostanol conversion in humans is variable: there are high converters showing almost complete cholesterol conversion and low converters with coprostanol representing less than 40% of the fecal neutral sterols content
[14]. Strains belonging to the genera of
Eubacterium and
Bacteroides have been identified as the prevalent cholesterol-reducing strains
[15][16]. Interestingly, coprostanol production is sex-dependent, with young women being better converters compared to young males
[17].
1.2. Trimethylamine/Trimethylamine N-Oxide (TMAO)
Foods such as meat, eggs, fish, brassica vegetables, peanuts, and soybeans are abundant in choline, betaine, phosphatidylcholine, lecithin, and L-carnitine
[18][19][20], which serve as dietary precursors of CM disease-associated compounds. Specifically, these nutrients are converted to trimethylamine (TMA) by particular intestinal bacterial strains endowed with TMA lyases. TMA is subsequently oxidized to trimethylamine N-oxide (TMAO) by the hepatic flavin monooxygenase 3 (FMO3)
[21]. TMA producers include: the
Firmicutes Anaerococcus,
Clostridium,
Desulfitobacterium,
Enterococcus, and
Streptococcus; the
Proteobacteria Dseulfovibrio,
Enterobacter,
Escherichia,
Klebsiella,
Proteus,
Pseudomonas, the
Actinobacteria Actinobacter, and
Citrobacter [22], while
Bacteroidetes are not TMA producers
[23]. Additionally,
Akkermansia,
Sporobacter,
Prevotella [23], and
Ruminococcus Gnavus, highly represented in GM of patients with atherosclerotic CAD, are great TMAO producers
[24].
Noteworthy, the TMAO precursor choline can be also produced directly by GM via phospholipase D (PLD) enzyme, thus confirming the important direct involvement of GM in TMAO production
[25].
TMAO represents a risk factor for CAD development as demonstrated by several preclinical and clinical data
[20]. In mice, TMAO levels increased upon dietary supplementation of choline and L-carnitine, prompting macrophage foam cell formation and development of atherosclerosis
[18][20]. The association of raised TMAO production and adverse CV outcome is also shown in numerous clinical studies
[26][27]. In particular, increased TMAO levels have been associated with an elevated risk of fatal and non-fatal myocardial infarction (MI) or stroke
[28]. In a large independent clinical cohort (
n = 4007), patients in the highest quartile of TMAO plasma levels had a 2.5-fold higher risk of a major adverse CV event than patients in the lowest quartile
[19]. Moreover, TMAO circulating levels predict 5-year mortality in patients with stable coronary artery disease
[27].
The mechanisms by which TMAO increases GM-mediated CV risk are numerous. TMAO influences lipid composition
[28], alters BAs transport, composition, and pool size, induces C-reactive protein production, fosters endothelial dysfunction, and increases serum levels of the proinflammatory LPS endotoxin
[29]. Moreover, TMAO enhances platelet responsiveness to multiple distinct agonists (ADP, thrombin, and collagen) by facilitating Ca
2+ release from the intracellular stores and inducing a pro-thrombotic effect in vivo
[30]. Finally, TMAO promotes platelet aggregation also by activating the toll-like receptor (TLR) pathways
[31].
1.3. Phenyl-Acetylglutamine (PAG)
Phenyl-acetylglutamine (PAG) is a recently identified, protein-derived compound produced by GM, and is considered a risk factor for atherosclerosis. It is obtained from the conversion of phenylalanine into phenylacetic acid performed by specific strains of GM. Phenylacetic acid is then conjugated with glutamine in the liver, generating phenylaceticglutamine (PAG)
[32]. PAG affects platelet responsiveness inducing thrombosis through adrenergic receptor activation
[32]. In the Malmo Offspring Study, Ottoson demonstrated that PAG was correlated with GM composition and associated with an increased risk of future CAD independently of other CV risk factors
[33].
1.4. Short Chain Fatty Acid Production (SCFAs)
Besides transforming the compounds introduced with the diet in pro-atherogenic mediators, GM can also produce metabolites with a protective role.
SCFAs are GM-derived metabolites produced by the fermentation of complex carbohydrates, mostly from fermentable fibers such as pectin, beta-glucans, guar-gum, inulin, and phospho-oligosaccharides, largely contained in fruit, vegetables, and whole grains
[34]. The most abundant SCFA is acetate (up to 75% of total SCFAs) followed by propionate and butyrate
[35]. Acetate and butyrate are produced by members of the
Bacteroidetes phylum, whereas butyrate is produced by species of the
Firmicutes phylum
[36]. In the specific case of Firmicutes, most gut bacteria representing this phylum are gram-positive and are able to produce several SCFAs, contributing to the protective CVD phenotype
[7].
SCFAs influence numerous processes such as host-microbe signaling, energy utilization, and control of intestinal pH, entailing effects on the GM composition and gut motility
[37]. SCFAs contribute to maintaining the intestinal barrier integrity by stimulating the production of mucin and regulating the expression of tight junction proteins
[38]. The efficiency of the intestinal mucosa barrier allows a reduction of the leakage of molecules and cells from the gut into the bloodstream and, in turn, participates in reducing the systemic inflammatory condition. Thus, the SCFAs have anti-inflammatory effects
[39].
SCFAs and, especially butyrate, act on specific plasma membrane receptors to mainly transduce inhibitory effects. For example, the interaction of SCFAs with receptors of immune response cells represses the activation of the nuclear factor NFκB and the activity of histone-deacetylases (HDAC)
[40][41]. These two mechanisms are responsible for stopping the proliferation of T lymphocytes, and for inducing apoptosis of activated T lymphocyte when SCFA concentration increases further
[42]. The same two mechanisms also blunt the release of TNF-alpha by granulocytes, monocytes, and macrophages after contact with bacterial membrane LPS
[43]. In addition, SCFAs activate regulatory T (Treg) cells, through HDAC inhibition, playing a regulatory or suppressive activity on inflammatory signaling
[40].
Since the SCFA receptors are widespread in many organs and cells such as intestinal epithelium, or adipocytes, the overall action of SCFAs lowers serum lipid levels though several routes: (i) by blocking cholesterol synthesis, (ii) by redirecting lipids towards the liver
[44], and (iii) by reducing the triglycerides accumulation in fat cells
[45]. Collectively, these SCFA-mediated processes have a protective role against the development of overweight, obesity, and CAD
[45]
1.5. Polyphenols
Dietary polyphenols are naturally occurring compounds present in food items such as vegetables, fruits, cereals, tea, coffee, dark chocolate, cocoa powder, and wine
[46]. The main groups of dietary polyphenols are phenolic acids, flavonoids, tannins, stilbenes, and diferuloylmethanes. The chemical composition of these molecules is extremely variable but presents common domains, i.e., hydroxylated aromatic rings or phenol rings
[47]. A large proportion of polyphenols remain unabsorbed along the small intestine and may accumulate in the large intestine (90–95%), where it is extensively metabolized by GM. In turn, the transformed polyphenols have the ability to modify GM growth and composition in a two-way relationship
[46]. For example, berberine, a polyphenol with poor oral bioavailability, significantly reduced atherosclerosis in high-fat-diet fed mice by stimulating the growth of
Akkermansia [48]. Along the same line, the ability of resveratrol to counteract the metabolic syndrome-related alterations, including derangement of glucose and lipid homeostasis, increase of fat mass, rise in blood pressure, low-grade inflammation, and oxidative stress, is mainly due to the regulation of GM composition, BAs biosynthesis, and TMAO production
[49]. Furthermore, GM converts polyphenols into secondary metabolites with potential health effects. This is the case of the lignin derivatives, such as enterodiol and enterolactone, that exert protective effects including a 30% reduction of all-cause mortality risk, along with decreased CVD mortality and non-fatal myocardial infarction
[50].
Therefore, when compared to a western diet, a polyphenol rich nutritional regimen, such as the Mediterranean one, must be preferred to increase the biodiversity of the GM and ensure advantageous effects for the health of the host (Figure 1).
Figure 1. Schematic representation of the effects of different diet regimens on gut microbiota composition with opposite repercussions on CM and CVD risk factors. PAG = phenyl-acetylglutamine, SBA = secondary bile acids, SCFA = short chain fatty acid, TMAO = trimethylamine N-oxide.
2. Sex Difference and GM Diversity: Bidirectional Cross Talk between Intestinal Microbial Composition and Sex Hormones
2.1. Sex Hormones Regulates GM Composition and Function
Like other members of the steroid hormones superfamily, SH act prevalently through the classical genomic mechanism that involves binding to specific nuclear steroid hormone receptors (SHR) transcription factors, including estrogen receptors (ER), progesterone receptors (PR), and androgen receptors (AR)
[51]. Besides the classical mechanism of action, sex steroids can act in the cells through the nonclassical or nongenomic mechanism of action, in most cases mediated by membrane receptors.
Through these pathways, SH may influence GM composition: (1) by a direct action on bacteria, or (2) indirectly, by affecting intestinal PH and motility, and by modifying the function of the intestinal epithelium and local immunological response, thus influencing the environment for bacteria growth.
2.1.1. Direct Effects of Sex Hormones on GM Profile
SH directly affect bacterial growth and induction of virulence factors. In particular, SH, by interacting with ER beta, (ERβ) modulate the bacteria metabolism
[52]. For instance, it has long been recognized that the uptakes estradiol and progesterone by the anaerobic bacteria
Prevotella Intermedius, favors bacterial expansion
[53]. In addition, progesterone and estradiol can act as surrogates of vitamin K, an essential growth factor for
Prevotella Intermedius [54] (Figure 2).
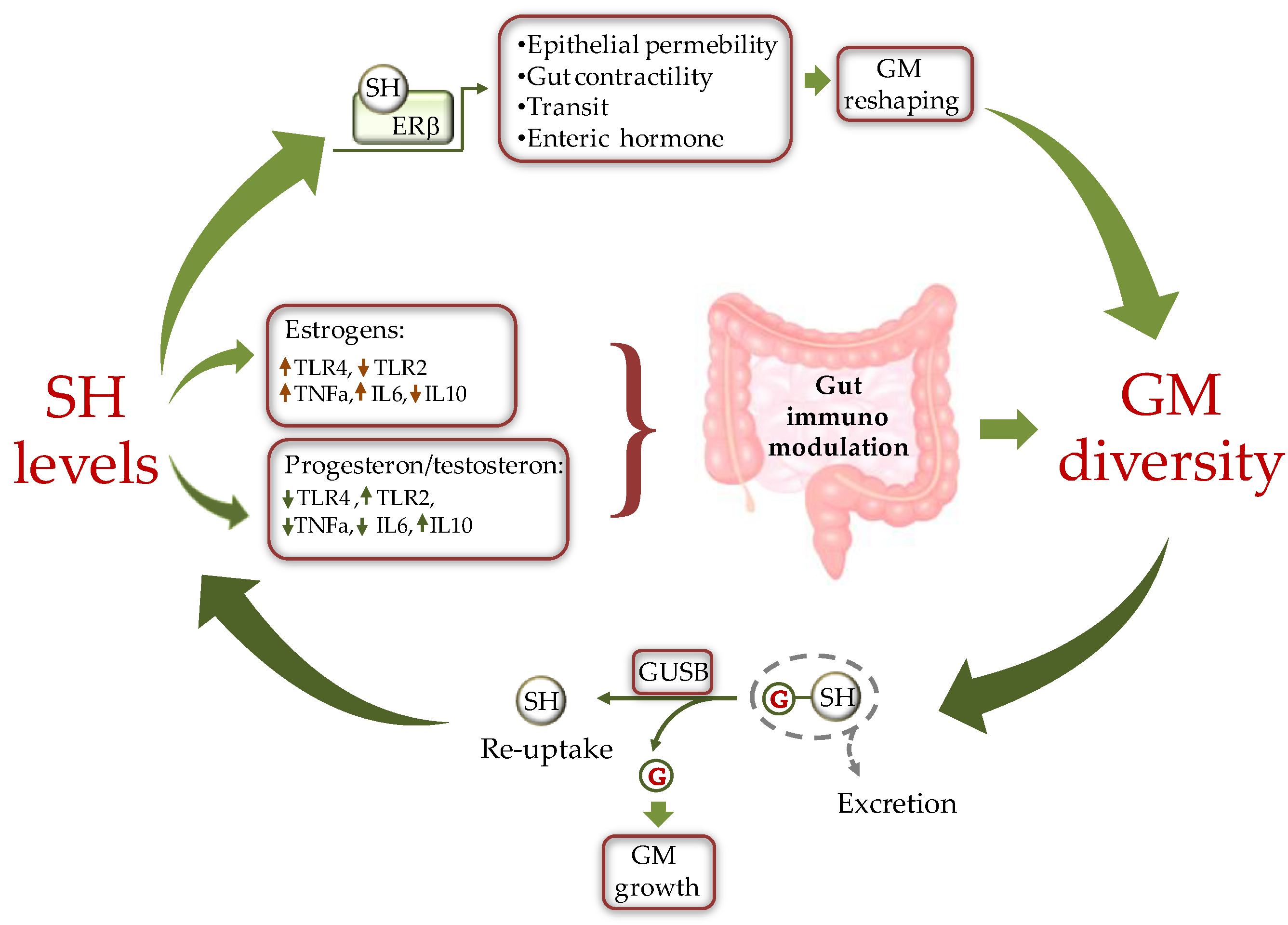
Figure 2. Schematic representation of the cross-talk between sex hormones (SH) and gut microbiota (GM). SH affect GM composition by modulating the intestine milieu via: i) a direct gene expression regulation in epithelial cells; and ii) by modulating the gut immune system through differential activation of TLRs. In turn GM influences SH circulating level by fine-tuning the balance between hormone excretion and reuptake. ERb = estrogen receptor beta, GUSB = β-glucuronidases; G = glucuronic moiety, IL = inteleukin, TLR = toll like receptor.
Sex steroids could also directly affect the composition of the GM by modifying substrate levels and energy production as the result of changes in bacterial beta glucuronidase (GUSB) activity (
Figure 2). Many gut bacteria synthesize GUSB enzymes. These proteins catalyze the release of glucuronic acid from host-derived molecules including SH, previously conjugated in the liver. The released carbon sources are then used by the intestinal bacteria as energy substrate to promote their own growth
[55].
2.1.2. Indirect Effects of Sex Hormones on GM Profile
Sex steroids may indirectly modulate the GM growth through activation of specific SHR on the intestinal colonic cells of the host
[56]. As a consequence, the intestinal abundance and localization of SHR subtypes is expected to greatly impact GM distribution, composition, and function.
ERβ is the most abundant ER in colon epithelial cells, followed by ERα. In accordance with its distribution, ERβ is involved in the organization and architectural maintenance of the colon epithelium
[57][58]. Indeed, Erβ-knockout mice exhibit a number of pre-pathogenic phenotypes, including disrupted cell-to-cell tight junctions and altered GM compared with wild-type mice, thus indicating a specific physiological role of ERβ in regulating the permeability of colonic epithelia
[57][58].
AR is more expressed in human colon rectal mucosa and in colon stromal cells where it contributes to the maintenance of intestinal homeostasis
[59]. The modified microenvironment might, in turn, affect the GM composition.
The classical ligand-dependent regulation of SHR activity by SH in the gastrointestinal cells also influences gut functions such as contractility, transit, or secretion of enteric hormones
[60], which in turn induce GM modifications (
Figure 2).
Numerous studies have evidenced a close connection between inflammatory state observed in several disease conditions and changes in the composition of GM
[61]. SH transcriptional activity influences GM composition by differentially affecting the local intestinal immune environment in a gender-specific way. Estrogens and androgens have opposite effects on the immune response. In particular, estrogens favor a hyperactive immune environment, while androgens (testosterone) have a suppressive effect on T cell proliferation, resulting in an anti-inflammatory condition
[62]. On the other hand, progesterone promotes the synthesis of anti-inflammatory cytokines and inhibits the production of their pro-inflammatory counterpart
[63].
A major mechanism linking SH, immune response, and GM deals with the integrity of the intestinal epithelial barrier
[64]. Decreased cohesion of the gut epithelium is associated with infiltration of gram-negative bacteria into circulation and activation of a peripheral inflammatory response by LPS, which may exasperate existing pathologies such as CM and CV disease
[54][65]. The interaction between certain GM strains and estrogens alters the integrity of the intestinal barrier and enhances cell-mediated and humoral immune response, natural killer (NK) cell cytotoxicity, and the production of pro-inflammatory cytokines such as interleukin 1 (IL-1), interleukin 6 (IL-6), and tumor necrosis factor alpha (TNF-α)
[64], thus favoring a proinflammatory phenotype.
As concerns the underlying pathway, in females there is an increased expression of genes involved in the pro-inflammatory TLR pathways. As evidenced by experimental studies, estrogenic treatment in mice increases cell membrane content of TLR4
[66]. Once activated, TLRs induce a pro-inflammatory immune environment that compromises gut permeability, causing translocation of gut commensals into the lamina propria where they can amplify pro-inflammatory responses
[54] (
Figure 2). In contrast, to estrogens, androgens, such as testosterone, decrease TLR4 and TLR2 expression in macrophage and down-regulate NK cells and TNF-α production while enhancing the production of the anti-inflammatory interleukin 10 (IL-10)
[67]. Ultimately, the repression of TLR pathways and antigen presentation by testosterone favors the integrity of the intestinal barrier
[67]. Similarly to testosterone, progesterone contrasts the activation of LPS-mediated TLR4 signaling
[68] and suppresses NK cell activity, thus fostering the integrity of the intestinal epithelium
[54]. Additionally, progesterone decreases gut permeability through upregulating occludin in human intestinal tissue
[69].
A recently identified pathway involving SHR, intestinal homeostasis, and GM diversity deals with the activity of the orphan nuclear estrogen-related receptor alpha (ESRRA) on mitochondrial function. This factor is critical to maintain mitochondrial biogenesis and macroautophagy/autophagy function in the gut. It has been demonstrated that ESRRA acts as a key regulator of intestinal homeostasis by ameliorating colonic inflammation through activation of autophagic flux and control of host GM composition. Thus, ESRRA contributes to intestinal homeostasis to protect the host from detrimental inflammation and dysfunctional mitochondria
[70].
In conclusion, changes in estrogens, progesterone, or androgens signaling could result in altered intestinal functions, such as contractility and transit, and in a local immune unbalance, which may influence the gender-specific GM composition by differently shaping the gut mucosal environment.
2.2. GM Composition Regulates Sex Hormone Levels
Just as GM is influenced by SH, in turn GM may be an important regulator of circulating levels of SH and SH metabolites
[71]. In fact, GM is able to produce hormones (e.g., serotonin, dopamine, somatostatine), and to regulate the host’s hormones homeostasis by inhibiting gene transcription (e.g., prolactin) or fostering conversion reactions (e.g., glucocorticoids to androgens)
[72].
The set of enteric bacterial genes coding for estrogen-metabolizing enzymes, is defined as “estrobolome”
[73]. As above anticipated, the bacterial GUSB influences the systemic estrogen metabolite (EM) profiles. Normally, in the phase I metabolic step, estrogens, and their metabolites are conjugated by the liver and excreted in the bile. Of note, glucuronidation of estrogens serves primarily a classical excretory role given that conjugated EM have a low affinity for the ERs and do not promote appreciable transcription. Once secreted within the intestine, EM can be de-conjugated by the GM GUSB, reabsorbed by the gut, and released into the bloodstream for distal action. Thus, depending on GM composition, the estrobolome favors deconjugation and promotes reabsorption of free estrogens into enterohepatic circulation, which in turn contributes to the host’s total estrogen amount
[54][65]. When this process is impaired through dysbiosis, the altered deconjugation process results in variation of circulating SH levels. For example, a reduction of bacterial GUSB activity may contribute to the development of pathological conditions such as obesity, metabolic syndrome, cardiovascular disease (CVD), and decline of cognitive function
[3][64]. On the other side, the increased abundance of GUSB-producing bacteria can induce hyperestrogenic pathologies. This condition results in increased free estrogens circulating levels, entailing diseases such as endometriosis and cancer
[74][75].
Increasing findings also indicate that male androgen levels are subjected to GM-dependent modulation. In studies on obese male mice, the treatment with lactic acid bacteria isolated from human milk induces growth of testes and increased serum testosterone levels
[76]. In addition, feeding the old male mice with purified microbes such as
Lactobacillus Reuteri restores the testosterone to the youthful level
[77]. Collectively, these results provide evidence that GM is able to affect testosterone production and testicular aging.
Although human androgen biosynthesis and metabolism have been extensively studied in the past, the exact underlying mechanisms still remain elusive. Traditionally, most androgen has been thought to be metabolized by the liver and subsequently excreted by the kidney
[78]. However, a recent study by Collden et al. pointed at the GM as a main regulator of androgen metabolism
[79]. In effect, similarly to estrogens, conjugated androgens are hydrolyzed in the intestinal tract via bacterial GUSB into free androgens for reabsorption. A reduced concentration of circulating androgen may induce androgen-related disease. As reported by Harada et al., the GM was largely involved in the CV risks associated with male hypogonadism, which could be prevented by antibiotic therapeutic strategies
[80][81]. In line with this notion, in a recent post hoc analysis, men with metabolic syndrome and low levels of high-density lipoprotein (HDL)-cholesterol evidenced an association between reduced testosterone concentrations and increased risk of subsequent CV events and death
[82]. This finding is confirmed by other epidemiological studies showing that low testosterone levels in men are negatively associated with the degree of carotid atherosclerosis
[83]. Finally, it is well-established that male hypogonadism in aging men is closely associated with an increased risk of metabolic syndrome and T2D
[84].
Besides the androgen deglucuronidation activity of GM, some specific microbial taxa, including
Butyricicoccus Desmolans,
Clostridium Cadaveris,
Propionimicrobium Lymphophilum,
Clostridium Scindens, and
Clostridium Innocuum, express classical steroid processing enzymes such as steroid-17, 20-desmolase, 20β-, 20α-, and 3α-hydroxysteroid dehydrogenase, or 5β-reductase, and possess the potential capability to directly metabolize steroid hormones
[85][86]. In addition, glucocorticoids can be converted into androgens via side-chain cleaving capacity of bacteria
[87][88]. Finally, to make the scenario of interactions between GM and SH even more complex, a recent work has shown the ability of some bacterial strains to retroconvert estrogens into androgens
[89].
Overall, the present evidence underlines a critical role of GM in estrogens and androgen metabolism, which deserves to be explored in more detailed studies.
This entry is adapted from the peer-reviewed paper 10.3390/ijms23137154