Innate lymphoid cells (ILCs) represent a heterogeneous population of non-B/non-T lymphocytes and they are defined mainly by three unique features: (1) their lymphoid morphology; (2) their lack of genetically rearranged antigen receptors; and (3) their deficiency of cell-surface markers expressed in other immune cell types, such as myeloid cells and dendritic cells.
Like all known lymphoid lineages, ILCs originate and develop from common lymphoid progenitors (CLPs) found within fetal liver and adult bone marrow (
Figure 1)
[22][23]. Based on their differential development trajectories and functions, the ILC family is categorized into five subsets: natural killer cells (NK cells, or “killer” ILCs), “helper” ILCs (ILC1s, ILC2s and ILC3s) and lymphoid tissue inducer (LTi) cells (
Figure 1)
[1][2][24]. Many current views regard ILCs as the innate counterpart of helper T cells, due to their strong similarities in the production and output of signature cytokines, and in the expression and production of several key transcription factors (TFs)
[25][26]. ILC1s mirror CD4
+ T helper (Th)1 cells, ILC2s mirror Th2 cells, ILC3s mirror Th17 cells, while NK cells represent the innate counterpart of CD8
+ cytotoxic T cells
[2][27]. Despite these similarities, ILCs’ unique epigenetic and transcriptional programs, as well as their crucial impact on health and disease, imply their nonredundant roles
[28][29].
NK cells were first discovered in mice and humans in 1975
[30]. They are derived from natural killer cell precursor (NKP) cells, dependent on and characterized by the expression of the TFs Tbx21 (T-bet) and eomesodermin (Eomes)
[31][32]. NK cells circulate in the bloodstream and are the counterpart of CD8
+ cytotoxic T cells. They react to tumors and intracellular pathogens, producing interferon-γ (IFN-γ), granzymes and perforin, to kill tumor cells or normal cells infected by the virus
[33][34]. ILC1s are the innate counterpart of Th1 cells and, resembling NK cells, are also involved in the immune response to tumor cells and intracellular pathogens, such as viruses and certain bacteria
[35][36]. There are some common features between ILC1s and NK cells, such as requiring either T-bet, Eomes, or both, to achieve development and expressing IFN-γ as their principal cytokine output
[37][38]. The difference is their discrepant developmental pathways and functional features. NK cells develop via NKPs, while ILC1s, like the other two “helper” ILCs, develop via ILCPs. In addition, ILC1s are tissue-resident cells and express only low levels of perforin, with less cytotoxicity
[3][39]. ILC2s are defined by the expression of TFs GATA-binding protein 3 (GATA3) and retinoic acid receptor-related orphan receptor α (RORα), as well as the output of Th2 cytokines, including interleukin (IL)-4, IL-5, IL-9, IL-13 and the epidermal growth factor amphiregulin
[40][41]. In addition to showing a response to parasitization
[42], ILC2s are also involved in tissue repair and metabolic processes by type 2 immune responses
[43][44][45]. ILC3s are the innate counterpart of Th17 cells. In mice, ILC3s rely on the TFs RORγt to develop and perform their functions and produce cytokines IL-17 and either IL-22, granulocyte macrophage colony-stimulating factor (GM-CSF, also known as Csf2), lymphotoxin, or a combination
[2][46][47][48]. ILC3s are abundant in mucosal tissue, where they perform the innate immune response to extracellular pathogens and develop immune tolerance to intestinal symbionts. Thus, ILC dysfunction may lead to inflammatory diseases in mucosal-related tissues. LTi cells originate from CLPs via LTiPs and are strictly dependent on RORγt
[49]. During the stage of embryonic development, LTi cells produce the cytokines of lymphotoxin, which play a key role in the formation of Peyer’s patches as well as secondary lymph nodes
[50][51].
3. ILCs in Diabetes Mellitus
Diabetes mellitus is a progressive and complex metabolic disorder, characterized by chronic hyperglycemia, caused by impaired insulin secretion and (or) utilization.
ILCs resident in AT have been proven to limit or promote the development of obesity and obesity-associated T2DM (Figure 2). In healthy lean individuals, AT is enriched with type 2 immune cells, such as ILC2s, eosinophils and alternatively activated macrophages (AAMs, anti-inflammatory or M2 macrophages), to maintain tissue homeostasis and support a metabolically healthy state. During the process of type 2 immunity in AT, ILC2 plays an integral role in communication and regulation, and are therefore indispensable regulators. On the one hand, ILC2s produce cytokines IL-5 and IL-13, promoting the recruitment and accumulation of eosinophils and AAMs to support AT remodeling and to restrict “type 1” inflammatory responses. On the other hand, ILC2s promote the beiging of white adipose tissue (WAT), contributing to an increasing of both the quantity and the performance of beige adipocytes in AT. Through the two known mechanisms above, ILC2s help with the maintenance of AT balance and protect from obesity-associated metabolic dysfunction, insulin resistance and T2DM. In the AT of obese patients, diet-induced obesity initiates the early production of IL-12, which results in selective proliferation and accumulation of ILC1s which requires the IL-12 receptor and STAT4 signaling. ILC1-derived IFN-γ is necessary to accelerate classically activated macrophages (CAMs, proinflammatory macrophages or M1 macrophages) polarization and contributes to obesity-associated insulin resistance. In addition, adipose ILC1s have also been demonstrated to promote AT fibrogenesis by increasing M1 macrophages and activating the TGF-β1/Smad3 signaling pathway. By recruiting and activating M1 macrophages and inducing AT fibrosis, adipose-resident ILC1s participate in and promote the progression of insulin resistance and obesity-associated diabetes.
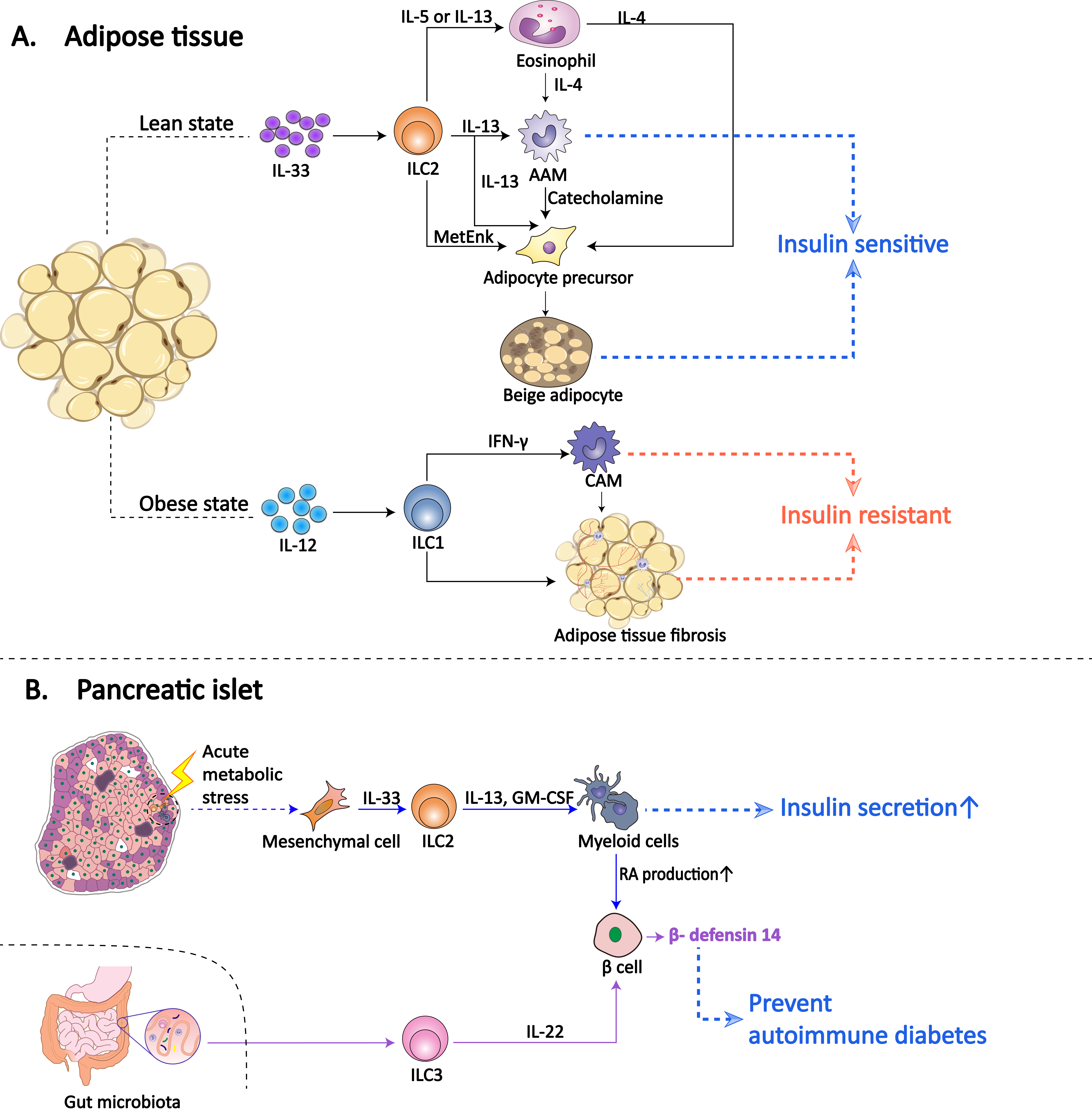
Figure 2. Role of ILCs in diabetes mellitus. A | ILCs in adipose tissue. In the lean state, IL-33 induces adipose-resident ILC2s to produce the cytokines IL-5 or IL-13, which support the recruitment and accumulation of eosinophils in AT. Eosinophils produce IL-4 to sustain and recruit AAMs. ILC2s produce ample IL 13 and may also directly contribute to AAM recruitment and maintenance. AAM byproducts, such as IL-10, contribute to adipocyte insulin sensitivity and protect against DM. In addition, IL-4, IL-13 and methionine-enkephalin peptides (MetEnk) and catecholamines, produced by eosinophils, ILC2s and AAMs, respectively, promote the proliferation and differentiation of adipocyte precursors into beige adipocytes. Beige fat biogenesis also promotes insulin sensitivity and prevents DM. In the obese state, while IL-12 promotes the selective accumulation of adipose-resident ILC1s. ILC1s drive CAM polarization by IFN-γ production and promote AT fibrosis, contributing to obesity-associated insulin resistance and DM. B | ILCs in pancreatic islets. In diabetic or obese states, the islets are also in an inflammatory background. IL-33 is produced by mesenchymal cells as a stress signal in islets. As the main IL-33-responsive cells in islets, islet-resident ILC2s stimulate the capacity of myeloid cells to produce RA, which in turn enhances insulin secretion in islet β cells and protects against DM. In the gut, the microbiota controls IL-22 expression by ILC3s within pancreatic islets through different pathways. ILC3-derived IL-22 induces islet β cells to produce β-defensin, preventing autoimmune diabetes.
A variety of substances that constitute the diabetic milieu, such as glucose and saturated fatty acids, stimulate the islet to produce various proinflammatory chemokines and cytokines and recruit and activate type 1 immune cells. Therefore, anti-inflammatory drugs for T2DM treatment are under development. Using immunofluorescence analyses, Dalmas et al. confirmed the existence of ILC2s located inside or in the periphery of islets in the mouse pancreas. Under conditions of islet inflammation in T2DM, proinflammatory factors induce mesenchymal cell-derived IL-33. Islet-resident ILC2s expressing the IL-33 receptor (IL-33R) are the major IL-33-responsive cells in islets. ILC2s increase the number of islet myeloid cells and elicit their capacity to promote retinoic acid (RA) production in a manner dependent on the secretion of IL-13 and GM-CSF. Ultimately, increased RA in turn enhances insulin secretion in islet β cells. The process above may be associated in part with the phenotypic plasticity shown by ILC2s in response to inflammatory signaling.
Islet-resident ILC3s have been found to play a role in protecting against autoimmune diabetes in mouse models (Figure 2). This function is mainly achieved by ILC3-induced mouse β-defensin 14 (mBD14) expression, and the activation of the former depends on gut microbiota. Defensin is a kind of antimicrobial peptide whose abnormal expression has been proven to be associated with diseases, including autoimmune diabetes. In the gut, the microbiota is known to control IL-22 expression in ILC3s through different pathways. On the one hand, by expressing aryl hydrocarbon receptor (AHR) ligands, some specific gut microbes can directly stimulate ILC3s to produce IL-22. On the other hand, other gut microbiota indirectly positively affects ILC3s via the induction of IL-23 secretion (a strong inducer of IL-22) by intestinal phagocytes. These pathways also work in islets to stimulate islet-resident ILC3s to secrete IL-22. In pancreatic islet, ILC3s are the major source of IL-22. ILC3-derived IL-22 induces islet β cells to produce mBD14, preventing autoimmune diabetes through the ILC3-IL22-mBD14 axis. In addition, ILC3s have also been found to produce GM-CSF and thus may play a partial role in regulating insulin secretion and protecting against T2DM.
4. ILCs (Mainly NK Cells) in Pancreatitis
Pancreatitis is an inflammatory disease of pancreatic tissue. Different etiologies, including pancreatic duct obstruction secondary to gallstones, alcohol abuse, as well as surgical trauma or pharmacological means, cause the dysfunction of cellular pathways and organelles, ultimately leading to acinar cell death and local and systemic inflammation. As a recently discovered immune cell group, the role of ILCs in pancreatitis has not been well studied. Current studies are mainly aimed at NK cells, but the knowledge gained is still relatively limited.
5. ILCs in Pancreatic Cancer
Pancreatic cancer (PC) is one of the most fatal malignant tumor whose incidence is only 14th among all cancers, while it remains the 7th most common cause of cancer death worldwide
[56]. It has become the third leading cause of cancer death in the United States, with the lowest 5-year survival rate among all cancers of 9%
[57]. Its characteristics of rapid progression, early metastasis and late diagnosis make PC the recognized “king of cancer”.
As crucial participants in innate immunity, ILCs undoubtedly play a role in the early stages of oncogenesis, in the formation of the tumor microenvironment, and in the whole process of tumor progression and metastasis. They sense malignant transformation and promote or inhibit tumor progression by producing an array of cytokines. To date, all known ILC subsets have been identified in PC but are found in different abundances. NK cells are the predominant ILC subset found in PC. They can prevent the growth of pancreatic tumors and induce remodeling of the tumor microenvironment, thus they have recently been targeted for tumor immunotherapy
[54][58][59]. In contrast, due the relatively late discovery of them, the inadequate studies on them and the lack of specific markers to identify them, little is known about noncytotoxic “helper” ILCs in pancreatic tumors. In fact, they may play a double-edged sword role in this disease (
Figure 3).
Figure 3. Role of ILCs in pancreatic cancer. ILCs may act as a double-edged sword in pancreatic cancer. In PC tissues, the frequencies of ILC2s and ILC3s are both significantly increased. Expanded by IL-33, ILC2s in PC potentially produce chemokine CCL5, which promote the recruitment and accumulation of CD103+ DCs in tumor tissues and further activate antitumor immunity in CD8+T cells. ILC2s express the PD-1, which restrains antitumor immunity. However, the PD-1 inhibition on ILC2 can be relieved by antibody-mediated PD-1 blockade, identifying ILC2s to be a potential, promising and brand-new target for anti-PD-1 immunotherapy. Unlike ILC2s, ILC3s promote the proliferation, metastasis and invasion of PC cells through IL-22/AKT signaling.
The presence of ILCs and ILC2s is detected in specimens from peripheral blood, pancreatic tissue and pancreatic tumor tissues of PC patients using flow cytometry, and higher ILC2 frequency is associated with longer survival
[60]. ILC2s may exert an antitumor efficiency in human PC. In KPC mice and orthotopic PC mice established with KPC cell lines, mouse ILC2s with a similar phenotype to human PC ILC2s are also identified, and display a characteristic tissue residency. Studies on the orthotopic mouse models of PC show that ILC2s in the PC microenvironment can activate antitumor immunity and act as targets of anti-PD-1 immunotherapy. Programmed death-1 (PD-1) is a T-cell coinhibitory receptor whose overexpression on tumor cells and tumor-infiltrating lymphocytes correlates with a poor disease outcome and tumor recurrence in many human cancers
[61][62][63][64]. Inhibition of the combination and interactions between PD-1 and its ligand PD-L1 can potentiate antitumor activity by enhancing T cell functions, which has been developed for cancer immunotherapy. Recently, PD-1 has also been found to be expressed in ILC2s and plays a negative regulation role in controlling cell proliferation and cytokine expression
[65][66]. Expanded by IL-33, ILC2s in PC potentially produce chemokine CCL5, which promote the recruitment and accumulation of CD103
+ DCs in tumor tissues and further activate antitumor immunity in CD8
+T cells
[60][67]. Similar antitumor activity has also been described in lung ILC2s, as IL-33-activated ILC2s produce cytokines IL-5 and IL-13, recruiting eosinophils and DCs to the tumor site, respectively, which limits tumor growth and invasion
[68][69]. However, PD-1 restrains the cell-intrinsic ILC2 functions described above. Further research suggests that antibody-mediated PD-1 blockade can release this PD-1 inhibition in tumor ILC2s, rather than in T cells, to activate antitumor immunity. That is, ILC2s act as tissue-specific enhancers to boost tumor immunity and amplify the therapeutic efficacy of anti-PD-1 in PC
[60]. In addition, IL-33 treatment is indicated to upregulate PD-1 expression in a fraction of tumor ILC2s; thus, a combination treatment of recombinant IL-33 and anti-PD-1 may maximally activate and enrich ILC2s in PC and enhance tumor control.
X. Xuan et al. analyzed the data of ILC frequency in pancreas and peripheral blood of PC patients and normal controls by flow cytometry, and they also observed the significantly increased levels of ILC2s and ILC3s in cancer tissues
[70]. This phenomenon is consistent with the findings of Moral et al.
[60]. By connecting the clinicopathological features of PC patients, it can found that the higher ILC3 frequency in tumor tissue is closely associated with tumor cell proliferation, vascular invasion and distant metastasis in human PC. IL-22, one of the major cytokines by which ILC3s perform their biological and pathological functions, has been found to be associated with the pathogenesis of many cancers, such as lung cancer, hepatocellular carcinoma, gastric cancer and colorectal cancer
[71][72][73][74]. A significantly elevated secretion level of IL-22 is also found in PC tissues, and ILC3s are its important source. ILC3s in the PC microenvironment enhance the potential of PC cells for proliferation, invasion and migration, by the combination of IL-22 with its cognate receptor IL-22R and the subsequent activation of the AKT signaling pathway, as is demonstrated by the in vitro experiments by X. Xuan et al.
[70].
Although some studies on the role of ILCs in PC have been undertaken, researchers understanding of this area is still vague. More data remain to be collected, and several important issues need to be addressed. First, PC mouse models constructed by injecting tumor cell lines or some chemical carcinogens have difficulty reproducing a physiological tumor microenvironment. Therefore, biopsy samples of human PC tissues are needed for detailed analysis of these ILC subsets. Furthermore, it is also necessary to develop better molecular tools or detection devices to quantitatively and qualitatively evaluate individual subsets of ILC. In addition, researchers need to explore the latent power of these cells not merely from their direct impact on PC cells but also from their ability to communicate with different components within the tumor microenvironment
[75], which may provide multiple insights into how to effectively manipulate and utilize ILCs for PC therapies.