Surface modification of electrospun products has been an attractive method for increasing multifunctionality and biocompatibility properties.
1. Introduction
Gelatin is a natural biopolymer obtained from collagen under controlled hydrolysis. It has well-known properties for drug delivery, wound healing, and tissue regeneration applications
[1][2][3][4][5][6][7]. Despite several advantages in biomedical engineering and regenerative medicine, gelatin has some major problems including melting at temperatures about or above 37 °C in water and hardening into gel at room temperatures. Therefore, specific pre-treatments are required for gelatin prior to applications in tissue engineering. Studies showed that gelatin can mimic different properties of collagen after the crosslinking approach with chemical reagents or physical processes
[1]. In this context, aldehyde-based chemicals have been proposed by some researchers despite their moderate toxicity in tissue regeneration studies. Therefore, researchers have explored bioactive, non-toxic, and natural compounds to stabilize gelatin biomacromolecules
[8]. Tannic acid is a plant polyphenol and a glucose derivative which is widely used as a natural reagent in the moisture, antioxidant, antimicrobial, antiviral, and anti-inflammatory products. Recent studies have approved that tannic acid can be effectively applied as a crosslinker for gelatin
[9][10].
Electrospinning is a simple and effective method for producing long, uniform, and fine diameter nanofibers. Resultant scaffolds from different nanofibers have shown specific properties such as high surface-to-volume ratio, high porosity, and long lengths. Notably, they have been used in various products such as artificial tissues, biomedical products, electronics, sensors, and nanocomposites
[11][12][13][14][15]. In the electrospinning process, different biomaterials can be utilized with effective mechanical properties in order to adapt and functionalize a biological environment
[13][14][15]. On the other hand, surface modification of electrospun products has been an attractive method for increasing multifunctionality and biocompatibility properties
[10]. A great number of studies demonstrated that different techniques can be applied for biofunctionalization of polymers including plasma, corona discharge, flame, photons, electron beam, ion beam, X-ray, and Gama-ray functionalization. However, plasma process of polymers has been known as a safe, cost-effective, and simple approach without affecting the bulk properties
[16][17][18][19].
2. Microscopic Assessment
The surface morphology of gelatin nanofiber scaffolds was assessed before and after treatment with air pressure plasma, using SEM and AFM methods.
Figure 1A,B indicates the results of SEM data for the untreated and plasma-treated gelatin nanofibers. There were no considerable changes on the surface, morphology, and the average diameter of nanofibers after plasma treatment. As a result of using acetic acid solvent, researchers previously found that several factors prevent bead-like patterns on electrospun nanofibers including electrostatic repulsion, surface tension, and viscoelastic properties. Therefore, researhers used optimized electrospinning conditions from the previous studies to generate smooth gelatin nanofibers
[9][10]. In the latest study on argon and argon–oxygen plasma-treated gelatin nanofibers, SEM did not indicate any surface changes which is in agreement with the results from air pressure plasma-treated nanofibers.
Figure 1. (A) SEM image of untreated gelatin nanofibers, (B) SEM image of atmospheric-air plasma-treated gelatin nanofibers, (C) AFM image of untreated gelatin nanofibers, (D) AFM image of atmospheric-air plasma-treated gelatin nanofibers.
For an accurate surface topography evaluation of scaffolds, researchers led a complementary AFM analysis (
Figure 1C,D). Researchers also extracted the output results from AFM analysis, and roughness parameters were illustrated in
Table 1. The surface of untreated fibers was smooth with an average RMS of 5.1 nm, as calculated from
Figure 1C. The RMS for atmospheric-air plasma-treated nanofibers (
Figure 1D) was 954.9 nm. A drastic increase in the surface roughness of nanofibers after plasma treatment can be resulted from several factors including the bombardment of energetic particles such as electrons, ions, radicals, neutrals, and excited atoms/molecules. On the other hands, chemical etching of polymer surfaces has been illustrated by plasma method due the bond breakage, chain scission, and chemical degradation
[20][21].
Table 1. Different parameters extracted from AFM analysis for the untreated and atmospheric-air plasma-treated gelatin nanofibers (standard deviations in parenthesis).
Samples |
Plasma Exposure Time (s) |
Maximum Peak Height, Ra (nm) |
Maximum Valley Depth, Rz (nm) |
Average Peak-to-Valley Height, Rq (nm) |
RMS (nm) |
Untreated nanofibers |
0 |
6.5 (0.02) |
276.8 (2.7) |
47.4 (1.1) |
5.1 (0.01) |
Plasma-treated nanofibers |
90 |
30.8 (0.1) |
479.8 (5.3) |
222.6 (2.5) |
954.9 (4.3) |
3. Water Contact Angle Properties of Nanofibers
One of the most important properties of biomedical scaffolds is their hydrophilic/hydrophobic functionalities
[22]. For this purpose, the contact angle (CA) of water droplets was measured on nanofibers using the device software.
Table 2 represented the results of CA for the untreated and atmospheric-air plasma-treated gelatin nanofibers. As expected, gelatin indicated excellent wettability in comparison with different hydrophobic biopolymers including polylactic acid and poly caprolactone, with a water CA of 20.65. This is owing to its hydrophilic nature
[23]. On the other hand, the water CA decreased to 11.8 for the atmospheric-air plasma-treated gelatin nanofibers due to the oxidation or attachment of polar groups
[24]. Several studies postulated that the introduction of oxygen-polar domains on the nanofiber surfaces can be conducted by the atmospheric-air plasma method, which can subsequently lead to a drastic change in their hydrophilicity
[22][23][24][25]. This observation is in good agreement with the ATR-FTIR and AFM results.
Table 2. The average water CA (°) of (A) untreated, (B) atmospheric air plasma-treated gelatin nanofibers.
Samples |
Water CA (°) |
Image from Camera |
A |
20.6 |
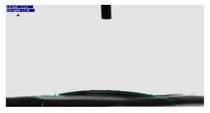 |
B |
11.8 |
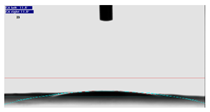 |
4. Effect of Atmospheric-Air Plasma on the Fibroblast Cell Culture
Fibroblast cells were put on the scaffolds and analyzed with inverted optical microscope along with SEM after 24 h.
As it can be seen from the inverted microscope (
Figure 2A with a magnification of 200×), fibroblast cells were flat. However, the microscopic examination did not reveal any major change in cell shape and adhesion to the atmospheric-air plasma-treated gelatin film (
Figure 2B). According to
Figure 2C from SEM, fibroblast cells were connected to the untreated gelatin film and their natural shapes were maintained after 24-h treatment with trypsin. On the other hand, there was no change in the shape of fibroblast cells on the plasma-treated gelatin film (
Figure 2D). Interestingly, it is found that the number of cells were increased on gelatin due to plasma functionalization. This result was in agreement with the study on argon and argon-oxygen plasma-treated gelatin nanofibers
[10]. Several studies stated that plasma modification has a critical role in improving the hydrophilicity of scaffold surfaces which can further enhance the proliferation and differentiation of osteoblastic cells
[26][27][28][29][30]. These researchers found that the number of oxygen atoms on tissue scaffolds was increased after atmospheric-air plasma treatment, thus the cell growth was improved.
Figure 2. Images of fibroblast cells on gelatin films, (A) inverted optical microscope image from the untreated gelatin film, (B) inverted optical microscope image from the atmospheric-air plasma-treated gelatin film, (C) SEM image from the untreated gelatin film, (D) SEM image from the atmospheric-air plasma-treated gelatin film.
This entry is adapted from the peer-reviewed paper 10.3390/biomedicines10030617