Electrocatalytic CO2 reduction (ECR) is an attractive approach to convert atmospheric CO2 to value-added chemicals and fuels. However, this process is still hindered by sluggish CO2 reaction kinetics and the lack of efficient electrocatalysts. Therefore, new strategies for electrocatalyst design should be developed to solve these problems. Two-dimensional (2D) materials possess great potential in ECR because of their unique electronic and structural properties, excellent electrical conductivity, high atomic utilization and high specific surface area.
1. Introduction
CO
2 is the main component of greenhouse gases which lead to environmental concerns, and its concentration has increased from approximately 280 ppm in the early 1800s to 410 ppm today
[1][2][3]. Without proper strategies to combat this problem, the steadily growing CO
2 emissions will arouse the increase in the global average temperature, loss of glaciers, rise of sea level and other climate issues
[4]. To reverse these negative courses, technologies such as carbon capture and storage (CCS) have been pursued
[5][6][7]. Despite tremendous efforts on CCS, including separating CO
2 from air or flue gas, storing the captured CO
2 is still a daunting challenge due to the risk of leakage, massive energy consumption, high cost and social acceptance
[8][9][10][11][12]. An alternative approach is to utilize CO
2 as raw material and convert it into value-added products, which approach has received extensive attention in the past decades
[13][14][15]. Therefore, the conversion of CO
2 has been regarded as a promising route for closing the carbon cycle and producing value-added chemicals and fuels.
The conversion of CO
2 can be realized by a variety of technologies such as thermochemical, electrochemical, photochemical, radiochemical and biochemical reactions
[16][17][18][19][20][21][22][23][24]. Among these, the reduction of CO
2 by electrochemical strategies is attractive and exhibits many advantages
[25][26]. For instance, the energy required for electrochemical reduction reactions can be provided by renewable energy such as solar, geothermal or wind energy. In addition, the reaction can be performed under ambient pressure and temperature. Moreover, the external voltages as well as electrolyte solutions can be adjusted to produce specific products.
In recent years, two-dimensional (2D) materials with novel geometric, electronic and optical properties greatly accelerated the development of catalysts. Two-dimensional materials, sometimes referred to as single-layer materials, are crystalline materials consisting of a single layer of atoms. These materials have promising applications in fields such as photovoltaics, semiconductors, electrodes and water purification. Two-dimensional materials can be categorized as either 2D allotropes of various elements or as compounds consisting of two or more covalently bonded elements. The elemental 2D materials generally carry the -ene suffix in their names, while the compounds have -ane or -ide suffixes. A wide range of 2D compounds, including metals, chalcogenides, group IV and V elements, oxides, carbides, nitrides, halides, hydroxides, hydrides, phosphates, phosphonates and covalent organic frameworks, have been investigated for various applications. Two-dimensional materials can exhibit semiconductive, insulative or even metallic behavior. Some of them with direct or indirect band gaps have great responses to ultraviolet and visible light. The electronic and optical properties of these 2D materials are tunable by adjusting the number of layers because of quantum confinement. Two-dimensional semiconductive materials display strong enhancements of Coulomb interactions among charge carriers and defects, contributing to longer-lived excitons compared to their bulk structures
[27]. The specific surface area of 2D materials is usually quite high and more active atoms can be exposed. The physicochemical properties of 2D materials, especially, can be effectively modulated by different engineering strategies, including heterostructure, doping, chemical functionalization, etc.
[28].
When employed as catalysts, 2D materials with nanosheets can show unique properties and excellent performance in catalytic reactions. Compared with conventional bulk materials, they have much higher specific surface areas and high percentages of bare surface atoms that can offer abundant active sites, boosting catalytic reactions. Note that the highly exposed surface atoms can potentially escape and form defect structures, leading to decreased coordination numbers of surface atoms, which are the preferential sites for the adsorption of reactants or intermediates. Similarly, the edge atoms of nanosheets with low coordination numbers can also exhibit novel catalytic properties. Therefore, 2D structures can promote the chemisorption of reactants and improve catalytic performance. A large class of 2D materials, such as noble metals, metal oxides, graphene, graphite carbon nitride (g–C
3N
4), transition metal dichalcogenides (TMDs), metal–organic frameworks (MOFs), etc., has been demonstrated to have great potential to catalyze CO
2 conversion reactions
[29][30][31][32][33][34].
2. Fundamentals of Electrochemical CO2 Reduction
2.1. CO2 Properties and the Reduction Products
CO2 is one of the most stable molecules with a strong O=C=O double bond, and its bond energy is higher than that of C–H and C–C bonds. During electrocatalytic processes, the breaking of the O=C=O bond requires a high activation energy. In addition, since ECR consists of multiple elementary steps, these electrocatalytic processes are more demanding than, for example, water-splitting reactions.
Electrocatalysts can effectively reduce activation energy, accelerate reactions or increase desired product selectivity in ECR. It should also dissociate water in solution to promote proton–electron transfer because the proton-assisted multiple-electron transfer can be beneficial for CO2 activation. Depending on the different pathways and the number of protons and electrons transferred, a range of products can be formed, including C1 products, such as carbon monoxide (CO), methanol (CH3OH), formic acid (HCOOH), methane (CH4), or C2 products, such as ethylene (C2H4), ethanol (C2H5OH) and others [35]. C2 products with higher energy densities are more valuable, but the synthesis of C2 products is more difficult than that of C1 products because of larger numbers of required protons during conversion.
2.2. Single-Electron vs. Proton-Coupled Electron Reactions
Research on ECR has been conducted ever since the early 19th century. ECR contains two half reactions occurring in the anode and the cathode, where different numbers of proton-coupled electron transfers occur. The standard electrode potentials of different reactions with reference to standard hydrogen electrons (SHEs) for different products in aqueous solutions are summarized in Table 1.
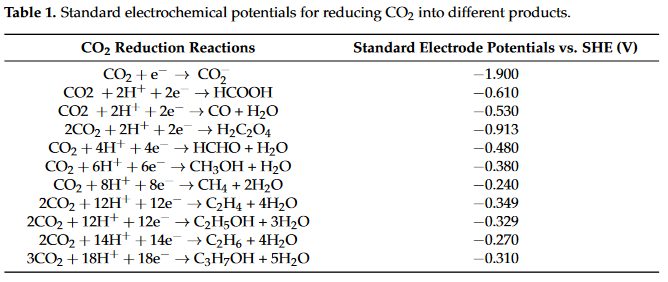
ECR starts by transferring a proton–electron pair to form carboxyl (*COOH) or formate (*OCHO) intermediate species [36]. These two intermediates can be further reduced by accepting different numbers of proton–electron pairs.
2.3. Factors Determining Product Selectivity
Factors such as the type of electrocatalyst (morphology, composition, chemical state and crystallographic structure), type of electrolyte (composition, concentration and pH), temperature, pressure and applied potential all influence ECR. For instance, solid and aqueous electrolytes have been used in ECR, exhibiting remarkable differences in efficiency
[37][38]. To date, NaHCO
3 and KHCO
3 solutions are frequently chosen as electrolytes because they can maintain the pH at the electrode surface. Meanwhile, the pH of the solution can affect the generation of by-products such as H
2.
ECR, with a more positive potential vs. SHE, is thermodynamically more favourable. In this regard, the conversion of CO2 to alcohol or hydrocarbon products should be thermodynamically more favourable than to other products, including CO, HCOOH and HCHO (Table 1).
2.4. Key Parameters for Evaluating ECR Efficiency
In experimental ECR study, there are several key parameters for evaluating electrocatalyst performance, including overpotential, current density, Tafel slope, FE, turnover frequency (TOF) and stability of electrocatalysts. The overpotential can be regarded as the energy needed to drive the reaction. In other words, the higher the overpotential, the higher the energy required for the reaction and the more difficult the reaction to be realized. Current density reflects the rate of electrochemical reaction, and the larger the current density, the faster the electrocatalytic reaction. The Tafel slope can be obtained by fitting the linear region of the Tafel curve. With the increase in overpotential, if the current density increases dramatically, the slope will be small. FE is regarded as the fraction between charges transferred to specific products and the total charges in the electrocatalytic process, accounting for the selectivity and efficiency of the reaction. Larger FE suggests less energy loss, and smaller FE indicates more energy loss. TOF is the catalytic activity of each site under a certain overpotential, indicating the intrinsic activity of catalysts. The stability of electrocatalysts can be evaluated by long-term use under a specific voltage.
3. Two-Dimensional Materials as Efficient ECR Electrocatalysts
Compared to bulk materials, 2D materials have a disordered state in their normal direction and a long-range order in the plane, showing a sheet structure. In addition, 2D materials can be exfoliated to single- or multiple-atom thickness, exhibiting interatomic covalent bonds in the plane. The physicochemical properties of 2D materials are different from that of bulk materials. The high atomic exposure rate, large specific surface area and flexible structure make them promising ECR catalysts.
3.1. Two-Dimensional Metallic Materials
Bulk metal catalysts in ECR have been investigated for decades
[39][40][41]. Metal electrocatalysts can be divided into two groups: noble metal (Au, Ag, Pt, etc.) and other earth-abundant transition metals (Co, Ni, Cu, etc.). Theoretical work has well disclosed that noble metals are better electrocatalysts compared with earth-abundant metals. Nevertheless, some intermediates, such as CO, could poison the active sites, which affects their stability. Large-scale application of precious metals is also not possible due to their high price and scarcity.
It has been revealed that precious metals with 2D nanosheet structures can enhance their electrocatalytic performance effectively and improve the utilization of noble metals
[42]. Huang et al. prepared a hexagonal Pd structure with 2.5 times higher electrocatalytic activity for the oxidation of formic acid compared to commercial Pd black
[43]. The high performance could be attributed to nanosheet structure with more active sites, better electronic structure and larger surface area, as well as high atom utilization. The results suggested that the current density increases with the decrease in particle size.
Moreover, multimetal composite nanosheets also exhibit excellent performance in electrocatalytic reactions because of the synergistic effect of different metals compared to single-metal electrocatalysts
[44][45][46].
Nonprecious metals, including Fe, Co, Ni, etc., have also attracted much attention in electrocatalysis due to low prices, abundant resources and promising electrochemical properties as well as tunable electronic structures
[47][48]. Studies have shown that Fe, Ni and Ti produce H
2 as the major product in ECR due to their low HER overpotential and strong CO adsorption capability. Sn, Pb and In have poor binding energy with
CO−2 intermediates and the final products are usually formate or formic acid. Moreover, Cu is the only metal catalyst that can produce C
3 hydrocarbons by a C–C coupling mechanism
[49].
Therefore, metal catalysts are effective for electrocatalysis due to their intrinsic activity and good conductivity. When the metal catalysts are synthesized with 2D nanosheets or other special nanostructures, the atom utilization, activity and selectivity could be improved, thus increasing electrocatalytic efficiency. Precious metals, such as Pd or Au, or multimetals with different ratios show excellent selectivity for CO generation. Nonprecious metals, such as Fe, Co and Ni, show poor ECR performance, while Sn, Pb and In can have good activity for the production of formate or formic acid. Particularly, Cu-based catalysts with different morphologies can exbibit different activities and selectivities for different products. Accordingly, the choice of metal, small nanosheet size and different morphology will have a positive effect on ECR activity and selectivity.
3.2. Graphene and Graphene-Based Materials
Generally, graphene is a single layer of graphite with a zero-band gap. It is a promising electrocatalyst for CO
2 reduction due to its high electron mobility, conductivity, unique electronic structure and large surface area. In addition, the high thermal conductivity can improve heat diffusion during the exothermic process, benefiting electrocatalysis. However, pristine graphene exhibits some drawbacks in ECR. For instance, the delocalized π bonding network of graphene negatively affects the adsorption of intermediates such as *COOH or *OCHO; therefore, graphene cannot effectively activate the CO
2 molecule and presents high-energy barriers for intermediate formation, leading to low ECR activity
[50].
Compared with nonmetal dopants, introducing single or multiple metal dopants to construct special graphene nanostructures could also exploit materials with excellent catalytic performance. Au nanoparticles embedded in graphene nanoribbon exhibit low overpotential, high FE for CO generation and excellent stability compared to amorphous carbon-supported Au nanoparticles, attributed to the change in electronic properties and the increase in active sites
[51].
Graphene-based composite materials also possess excellent conductivities and larger surface areas compared to pristine graphene. Huang et al. synthesized N-doped graphene monolayer-coated Sn foil, showing excellent flexibility with a high FE of 92% for formate at −1.0 V vs. RHE
[52]
For graphene-like materials, graphitic carbon nitride (g–C
3N
4) typically exhibits good chemical and thermal stability under ambient conditions. However, poor conductivity and less active sites limit its potential application in ECR. Strategies, including doping metal and constructing composite, have been developed to increase the activity of g–C
3N
4. Metal atoms can effectively modulate the electronic structure of g–C
3N
4, thus improving the catalytic activity. The g–C
3N
4 nanosheet and multiwalled carbon nanotubes composite have been shown to exhibit excellent stability and good electrocatalytic performance for CO
2 reduction to CO
[53]. The electrocatalytic activity of the composite arises from the C–N bonds, and the high conductivity allows numerous electrons to transfer rapidly to the C–N sites.
3.3. Two-Dimensional Transition Metal Dichalcogenides
Transition metal dichalcogenides (TMDs), such as MoS
2, MoSe
2 and WS
2, have the general formula MX
2, where M is a transition metal atom and X represents a chalcogen atom. The layers of X–M–X are stacked together by the van der Waals interaction. This is another class of 2D materials with exceptional physical and chemical properties to be applied as electrocatalysts
[54]. The large surface area of 2D TMDs can provide more active sites for reactions such as CO
2 reduction and water splitting
[55][56]. DFT calculations suggested that the excellent catalytic properties for metal-terminated edges of 2D TMDs can be attributed to its metallic character and high
d-electron density
[57]. It has been confirmed that intermediates, such as COOH and CHO, prefer to adsorb on MoS
2 and MoSe
2 edges than the bridge S or Se atoms, and CO species adsorb selectively on the metal atoms during ECR
[58][59]. Four different TMD materials, MoS
2, MoSe
2, WS
2 and WSe
2, have been investigated as ECR catalysts; of which, WSe
2 nanoflakes exhibit the highest activity at an overpotential of 65 mV with an exceptional current density of 330 mA/cm
2 and an FE of 85% for CO generation, surpassing other electrocatalysts
[60]. The excellent performance can be attributed to WSe
2 nanoflakes’ much lower charge transfer resistance and low work function. DFT calculations showed that the formation of CO is kinetically favorable.
3.4. MOF Materials
MOFs are a new type of porous material with metal ions or clusters coordinated with organic ligands. Due to their high porosity, large specific surface area and flexible structure, MOFs have been applied in electrocatalytic research recently
[34][61]. However, some disadvantages, such as poor conductivity and less active centers, hamper their electrocatalytic activity. Studies have shown that low-dimensional MOFs can achieve high electrocatalytic performance because MOF nanosheets can expose more active atoms and exhibit excellent electron transfer. Moreover, the metal atoms, ligand and the connection of metal centers with ligands also play vital roles in the electrocatalytic activity of MOFs. Kornienko et al. synthesized a thin film cobalt–porphyrin MOF on a carbon substrate. The FE of this sample was 76% at −0.70 V vs. RHE for CO
2 conversion to CO. In addition, it possessed a low Tafel slope of 165 mV/decade and the electrocatalytic stability was excellent
[62]. A Zn MOF synthesized by ionic exchange of Zn nodes with adsorbed Ni salts achieved an FE of 71.9% for CO at 10.48 mA/cm
2 under the potential of −0.89V vs. RHE
[63].
3.5. Metal Oxide Nanosheets
Metal/metal oxide composites and oxide-derived metal have also been developed as ECR electrocatalysts with high performance
[64][65][66]. Nevertheless, metal oxides still suffer problems such as instability and poor conductivity. It has been demonstrated that low-coordinated surface metal cations can be beneficial for CO
2 adsorption during ECR. First principles calculation revealed that both the density of states (DOS) and the charge density around the conduction band edges can be greatly enhanced
[67]. Therefore, faster carrier transport along the 2D ultrathin layer during ECR can be expected.
3.6. Two-Dimensional Materials Incorporated Single-Atom Catalysts
Single-atom catalysts (SACs) have enormous advantages in electrocatalysis in terms of 100% atom utilization and intriguing electronic structures
[68][69]. The traditionally supported nanoparticles or clusters exhibit structural irregularities on the nanoparticle surface. The surface reactivity has a close relationship with surface free energy, and the ratio between fully coordinated surface atoms and the number of vacancies distinguishes the reactivity of atoms with identical chemical compositions but different positions
[70][71][72]. Therefore, the conventional clusters with different surface reactivities can result in poor product selectivity. SACs are different from clusters because the surface free energy is homogeneous and the number of vacancies in the nearest neighbors is the same. Thus, SACs can show good stability and special intrinsic activity
[73]. Single-atom catalysts exhibit uniform active sites as ideal catalysts; however, one major challenge for SACs is synthesis because SACs are unstable and can agglomerate quickly due to high surface energy. Furthermore, the supported structures in the neighboring environment increase the heterogeneity of SACs’ active sites, and thus uniform activity cannot be easily achieved. However, 2D materials can be utilized as supports to improve the uniformity and performance of SACs in electrocatalytic reactions
[74][75]. Studies have evidenced that 2D materials, such as MoS
2, graphene and MXene, could stabilize the SACs and maintain their single-atom state
[76][77][78][79][80][81][82].
4. The Design of Electrolyzers
Numerous ECR studies are based on H-cells because they can be used conveniently to evaluate the activity of catalysts due to their easy operation and adaptability to different electrode materials and configurations
[83][84]. A typical H-cell electrolyzer contains two chambers that are separated by an ion exchange membrane. The counter electrode is in the anodic chamber, whereas the working electrode and reference electrode are in the cathodic chamber. During the ECR process, CO
2 is first dissolved in the liquid catholyte, such as KHCO
3 and NaHCO
3, and then transferred to the surface of the working electrode where ECR occurs. However, the thick diffusion layer and low solubility of CO
2 in water limit CO
2 mass transport to the catalyst surface, impeding its practical applications
[85].
Commercial ECR application requires continuous operation to realize high production efficiency. A microfluidic reactor for ECR has been designed where the electrolyte can continuously flow through the electrolyzer
[86][87]. The cathode and anode are separated by a very thin channel that is filled with flowing electrolytes. CO
2 is supplied from the back of the cathodic gas diffusion electrode (GDE), and ECR occurs when CO
2 reaches the surface of catalysts. The operation parameters, such as the composition and pH of the electrolyte, can be adjusted, which promotes ECR rates. GDEs are high-surface-area and porous electrodes. A typical GDE consists of a catalytic layer, a gas diffusion layer and a gas flow field. The structure and composition of GDE layers play a vital role in the transport of reactants and products because the transport processes influence the accumulation or depletion of intermediates on the catalysts, which determine ECR performance
[88][89]. Lim et al. prepared Sn electrocatalysts with dense tips (SnDT) GDE, showing formate productivity of 65 mg h
−1. It was stable for 72 h without changes in FE or current density
[90]. A 2D nanosheet Cu-loaded GDE achieved the highest partial current density of 131 mA cm
−2 for acetate production
[91]. Although high current density was realized, the microfluidic reactor still suffered product crossover, where the reduction of oxygen diffused to the cathode and the reoxidation of cathodic products diffused to the anode, decreasing the overall energy efficiency and productivity.
This entry is adapted from the peer-reviewed paper 10.3390/catal12020228