2.1. Reproductive Cycles in Humans and Rodents
Rodent models are widely used in order to uncover molecular estrogen interactions in energy homeostasis. Therefore, it is worthwhile to briefly review woman and rodent reproductive cycles, noting their similarities and differences (Figure 1).
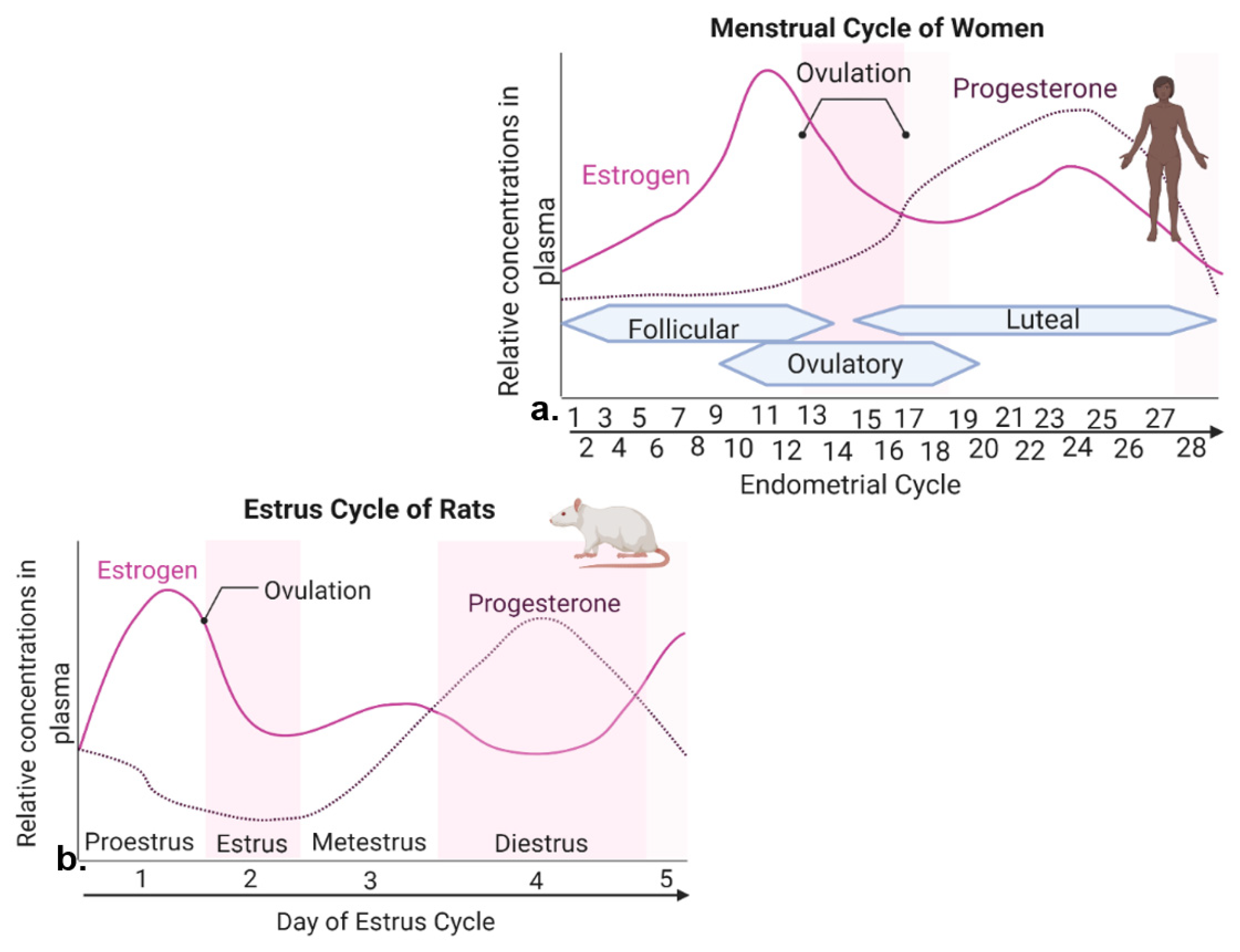
Figure 1. Levels of estrogen and progesterone during woman menstrual cycle and rodent estrous cycle. (a) The menstrual cycle of women takes place in ~28 days and consists of three prominent phases. The follicular phase is defined as the beginning of menstruation when shedding of the endometrium and bleeding occurs. During the beginning of this phase, estrogen and progesterone levels are low. Bleeding marks the start of a slight increase in follicular stimulating hormone (FSH) levels. FSH levels decrease and one follicle proceeds to develop, producing estrogen. The ovulatory phase is marked by a surge in luteinizing hormone (LH) and FSH, with ovulation occurring ~16–32 h post surge. Estrogen levels start to decrease, and progesterone increases. During the luteal phase, FSH and LH decrease, and the corpus luteum forms, which produces progesterone. Estrogen levels remain high, reaching its second largest peak. If fertilization does not occur, the corpus luteum degenerates, no longer producing progesterone, and estrogen levels decline, eventually causing the breakdown of uterus endometrium lining and the start of a new follicular phase. (b) The estrous cycle of rodents lasts ~4–5 days. Similar peaks of estrogen and progesterone are seen in menstrual cycle of women and estrous cycle of rats.
In women, the reproductive cycle is the menstrual cycle, also referred to as the endometrial cycle, which occurs ~28 days and consists of three prominent phases known as follicular, ovulatory, and luteal phases
[18] (
Figure 1a). The follicular phase distinguishes the beginning of menstruation, when shedding of the endometrium and bleeding occur
[18]. During the beginning of this phase, the levels of estrogen and progesterone are low. Bleeding marks the start of a slight increase in follicular stimulating hormone (FSH) levels, which causes the development of follicles in the ovaries
[18]. FSH levels then decrease and only one follicle proceeds to develop, which produces estrogen
[18]. The ovulatory phase is marked by a surge in luteinizing hormone (LH) and FSH, causing ovulation (i.e., egg release) ~16–32 h post surge
[18]. Estrogen levels start to decrease, and progesterone increases. During the luteal phase, levels of FSH and LH decrease, and the follicle forms into a corpus luteum, which produces progesterone and estrogen
[18]. Estrogen levels are increased, reaching the second largest peak. High levels of estrogen and progesterone cause the uterus lining to thicken to prepare for possible implantation of a fertilized egg
[18]. If fertilization does not occur, the corpus luteum degenerates, no longer producing progesterone, and as hormone levels decline, they eventually cause the breakdown of uterus endometrium lining and the start of a new follicular phase
[18].
The rodent form of the reproductive cycle is called the estrous cycle
[19], which occurs over a period of ~4–5 days. It consists of four main phases: proestrus, estrus, metestrus and diestrus
[19] (
Figure 1b), which correspond to three phases of the human menstrual cycle
[19][20]. Notice that during both human and rodent cycles, as in many mammals, there are two large peaks in estrogen and one peak in progesterone levels (
Figure 1)
[18][19]. Specifically, in both rodents and women, the highest peak of estrogen proceeds ovulation
[18][19]. The estrous cycle of rodents begins with proestrus, which is akin to the follicular phase in women that marks menstruation
[19][20]. During proestrus, there is a rise in circulating estrogen and a small surge in prolactin, along with a rise in FSH and LH levels
[19]. Proestrus is followed by a sharp decrease in estrogen levels and the start of ovulation, marking the estrous phase of rodents
[19]. After estrus, metestrus and diestrus occur next, and are homologous to the early and late secretary phases of the human endometrial cycle, in which the progesterone peak takes place
[19][20] (
Figure 1). In summary, naturally cycling female rodents are avidly used laboratory models of women, with regular reproductive cycles across which similar feeding behavior and metabolic changes are witnessed in response to hormone fluctuation.
2.2. Energy Homeostasis across Female Reproductive Cycles
The reproductive cycles and related fluctuating levels of estrogens affect caloric intake and macronutrient selection differently. In many species including humans, caloric intake changes across reproductive cycles due to changes in meal size, with females eating the most calories when estrogen levels are low during metestrus, and eating the fewest calories when estrogen levels are high immediately prior to ovulation
[17].
Interestingly, suppression of caloric intake by estrogen ceases when palatable food choices are offered alongside regular diet in female rhesus monkeys
[21]. Food choice and macronutrient intake are also affected by the menstrual cycle in women. Self-assessed food craving and macro- and micro-nutrient intake in 259 healthy women was analyzed for a period of two complete menstrual cycles
[22]. During the late luteal phase when estrogen levels are at their lowest level prior to menstruation, appetite and food craving for chocolate and sweet and salty flavors were higher, comparing with follicular and ovulatory phases when estrogen levels increase and peak during late follicular phase and beginning of ovulation
[22]. Interestingly, total protein intake and percentage of caloric intake from protein were greater during the mid-luteal phase with the second peak of estrogen levels, comparing with the ovulatory phase following the first and higher peak of estrogen (
Figure 1a)
[22].
In summary, the relationship between circulating hormone levels, feeding behavior, and energy metabolism is complex, and estrogen effects are dynamic across the female cycles in women and experimental animals.
2.3. Female Cycles Regulated by Estrogen
Estrogens plays critical roles in regulating great variety of physiological and behavioral functions. In females, the majority of estrogens, predominantly of the type 17β-estradiol (17β-E2) are synthesized in the periphery (i.e., ovaries) from cholesterol via the steroidogenic pathway involving a series of biochemical reactions
[23]. Estrogen steroidogenesis also occurs in Leydig and germ cells of the testis in males, although levels of produced estrogen are relatively low
[24]. In both males and females, gonadotropin releasing hormone (GnRH) from the hypothalamus triggers the anterior pituitary to secrete gonadotropins LH and FSH, which act on the gonads to stimulate steroidogenesis and gametogenesis
[25] (
Figure 2). In females, ovarian 17β-E2 synthesis and release from the ovaries negatively and positively regulates GnRH release in a pulsatile manner, although many effects of CNS-derived neuroestradiol on GnRH have been found to be more responsible for regulating GnRH (
Figure 2)
[25][26][27][28].
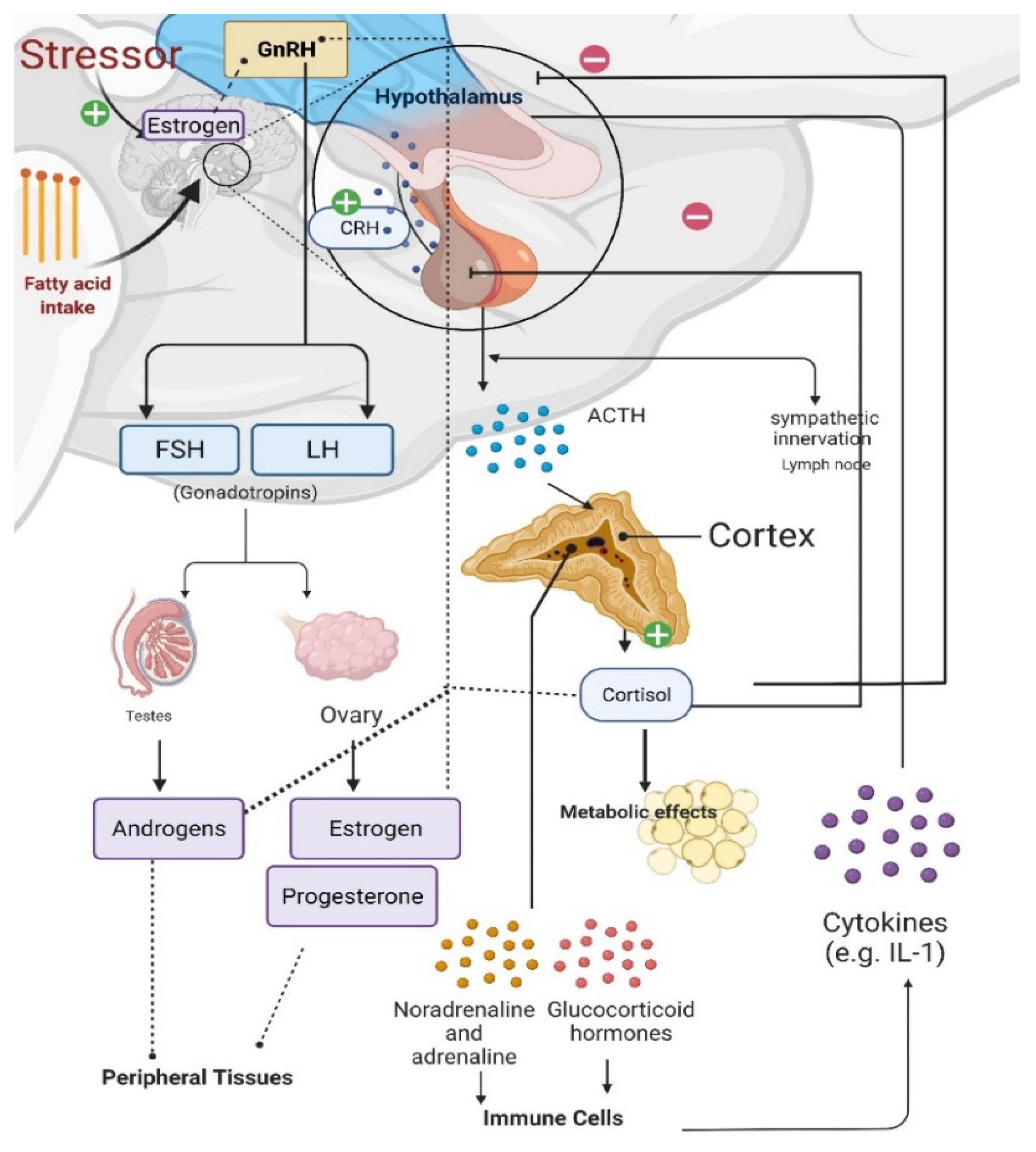
Figure 2. Effects of stress on the gonadal axis, immune cells, and metabolism. Gonadotropin releasing hormone (GnRH) release from the hypothalamus causes the anterior pituitary to secrete gonadotropins, luteinizing hormone [LH] and follicle-stimulating hormone [FSH]), which stimulate gonadal steroidogenesis. Estradiol synthesis and release from the ovaries regulates GnRH release. Estrogens can also be synthesized in the brain of males and females from androgens via aromatase in neurons; and estrogens can be synthesized in and have various effects at peripheral tissues. When the hypothalamic–pituitary–adrenal (HPA) axis is stimulated, corticotropin releasing hormone (CRH) from the hypothalamus triggers adrenocorticotropic hormone (ACTH) release from the anterior pituitary and increased cortisol production in the adrenal glands. Cortisol provides negative feedback to both the hypothalamus and pituitary; however, prolonged stress impairs this negative feedback regulation, causing long-lasting psychological and metabolic maladies. Prolonged fatty acid intake causes proliferative cytokines in the hypothalamus of males, which is protected in females.
Prolonged stress and increased glucocorticoid levels induce immune responses, elevate circulating cytokine levels, and dysregulate GnRH expression
[25]. Therefore, the HPA axis, the hypothalamic-pituitary-gonadal (HPG) axis, and immune cells all interact with each other (
Figure 2)
[29][30]. During the HPA axis response to stress, corticotropin releasing hormone (CRH) is released from the hypothalamus, causing the release of adrenocorticotropic hormone (ACTH) from the anterior pituitary and subsequent production of cortisol (in rodents)/corticosterone (in humans) from the adrenal cortex
[29][31]. Sympathetic activation during stress also regulates energy metabolism along with cardiovascular and pulmonary responses via sympathoneural (i.e., sympathetic innervation) and sympathoadrenomedullary (i.e., noradrenaline and adrenaline) systems (
Figure 2)
[32].
Major sex differences exist in stress-induced and stress-related metabolic disorders or disease states, pointing to differential interactions between the HPG axis, HPA axis, and immune cells
[29][33][34]. For example, prolonged HFD feeding causes inflammation with elevated proinflammatory cytokines in the hypothalamus, hippocampus, and cortex of males but not females
[35]. A recent experiment underlying female metabolic protection against HFD feeding has demonstrated peroxisome proliferator-activated receptor gamma coactivator 1 alpha (PGC-1α) as a regulator of ERα transcription
[35]. Specifically, chronic HFD exposure for 16 weeks causes increased proinflammatory cytokines, but reduced PGC-1α and ERα transcription, in the arcuate nucleus of the hypothalamus (ARC) of male mice, causing a metabolic dysfunction phenotype of myocardial complications. Conversely, both PGC-1α knockout (KO) and ERα overexpression resulted in protection from these metabolic complications
[35]. PGC-1α effects are likely to be upstream of ERα. Even in male mice, ERα can be protective against HFD feeding-related metabolic disorders. Novel regulators of ERα are still being discovered. Future studies that delve into the molecular details and underlying mechanisms of such sex differences await discovery.