Figure 1. (
A) CuNPs-graphene/Cu bioelectrode fabrication steps and its amperometric response to glucose injection
[2]; (
B) Synthesis procedure of Cu/Ni-EG/pNi, and the respective amperometric response to glucose injection
[5]. (
C) TEM images and amperometric response of Ag vertically deposited onto 3D nickel foam graphene and its amperometric response to glucose injection
[6]; (
D) Synthesis procedure of the CuFe-O/graphene onto nickel foam substrate and its amperometric response to glucose injection
[7] and (
E) Ni
2+ and Co co-reduction onto ITO for the Ni/reduced graphene oxide (rGO)/indium tin oxide (ITO) synthesis and its amperometric response to glucose injection
[8].
2. In-Situ Growth of Metallic Nanoparticles into Graphene Nanosheets
The use of graphene material as support enhanced the electrocatalytic activity, of the non-precious metals, receiving a lot of attention for glucose sensing
[9][10][11]. However, the uniform dispersion of them onto graphene support, without increasing the electrode’s internal resistance and at the same time acquiring a high catalytic performance, remains a challenge. According to many research groups
[12][13][14][15][16][17][18][19][20], an in-situ growth of the metallic nanoparticles inside graphene (or its derivatives) matrix, ensures homogeneous distribution of the nanoparticles and enrichment of the electron transport efficiency. Therefore, the formation of an inside nanostructured matrix with graphene (metal-graphene matrix composites), enhances significantly the electrocatalytic activity towards GER.
One of the most common approaches that is adopted when in-situ fabrication synthesis route is followed, is the application of metallic organic frameworks (MOFs) to the role of the precursor. The MOFs are being formed by the combination of inorganic element (metal element) being surrounded by organic linkers. Specifically, they own a cage-like structure which imparts its crystalline porous structure and an extremely huge internal surface area. Those special characteristics along with their functionable structures, chemical tunability and high compatibility, have make MOFs very attractive materials for a variety of applications
[21].
A characteristic example of in-situ growth of 2D MOF onto electrochemical EG surface synthesis method is depicted in
Figure 2B
[16]. Aiming at developing a high sensitive and stable NiCo-MOF-EG electrode for glucose sensing, Liu et al.
[16] followed an in-situ strategy. The MOF nucleation happened preferentially giving very thin nanosheets, as it is shown in
Figure 2B, via an easy and fast procedure. The special 2D MOF interconnected porous structure offered a very high specific surface area which facilitated the electrons transfer via metal ion to glucose molecule.
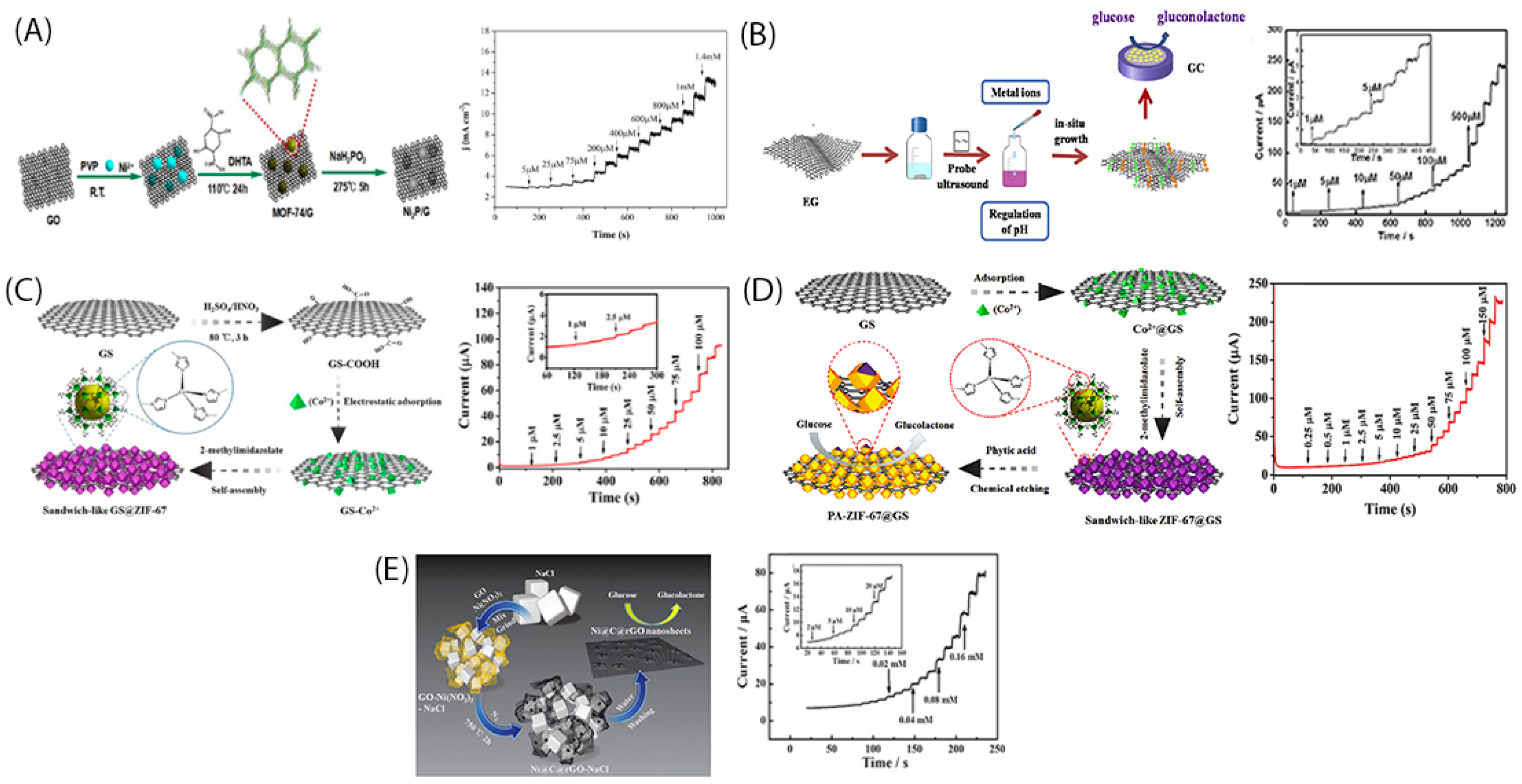
Figure 2. (
A) In-Situ synthesis procedure of Ni
2P/graphene and the respective amperometric response to glucose injection
[15]; (
B) In-Situ synthesis process of a NiCo-MOF-exfoliated graphene electrocatalyst and the respective amperometric response to glucose injection
[16]; (
C) In-Situ synthesis of graphene@ZIF-67 heterostructure and the respective amperometric response after the glucose injection
[17]; (
D) In-Situ growth of MOF into physically exfoliated graphene, functionalized the used MOF (ZIF-67) using phytic acid
[22] and (
E) In-Situ synthesis Ni@C@rGO nanosheets and the resulting amperometric response to glucose detection
[23].
3. Laser-Induced Graphene-Based Electrode Synthesis and Functionalization
Laser induced method for graphene synthesis and functionalization is very promising
[24]. Using laser tools, the synthesis and functionalization processes are accurately controllable, cost much less than other methods that use chemical agents, also allow the direct growth and functionalization of graphene onto the desired substrate. Moreover, laser methods have great reproducibility since the synthesis process is controlled by the laser operational characteristics, such as wavelength, energy density, etc, and not by chemicals. One way that has been followed by research groups
[25] to overcome this challenge is the creation of surface (or structural) defects using more controllable techniques, such as with the use of laser.
It has been found
[26] that under the appropriate functional conditions, the laser-engraved defects can be in balance with electrical conductivity. Thus, the created channels and pathways make much easier the charge and ions transport leading to faster response and higher sensitivity of a sensor
[27]. Another important advantage of the laser induced graphene electrodes, is the on-site construction of them onto flexible substrates/platforms; in combination with the fact that the raw materials are of lower cost without requiring any post-treatment steps, it seems to gain significant ground as a synthesis strategy. Following this synthesis method the degree of functionalization and the functional groups (-OH, -COOH and others) that are created depend on the operational characteristics of laser, such as wavelength, energy density, etc.,
[24].
Characteristically, recently, Zhang et al.
[28] constructed a 3D porous LIG (Laser induced graphene, with flake shape) electrode deposited onto a flexible substrate for glucose detection, having PI as polymeric substrate and using the produced gas through carbonization process (
Figure 3A). Then, Cu nanoparticles were deposited onto LIG by a substrate-assisted electroless deposition process
[29]. The resulting graphene nanosheets owned crumples and curves along with small holes, while a lot of graphene edges were present at the laser induced substrate. The graphene ‘defects’ were the channels for the electrons (being provided by the metal) delivery via the metal-carbon ‘bond’ and so the key for glucose electrooxidation reaction evolution. The sensitivity of the Cu-LIG nanoelectrode was estimated 495 μAmM
−1cm
−2 and the detection limit, 0.39 μM (
Figure 3A).
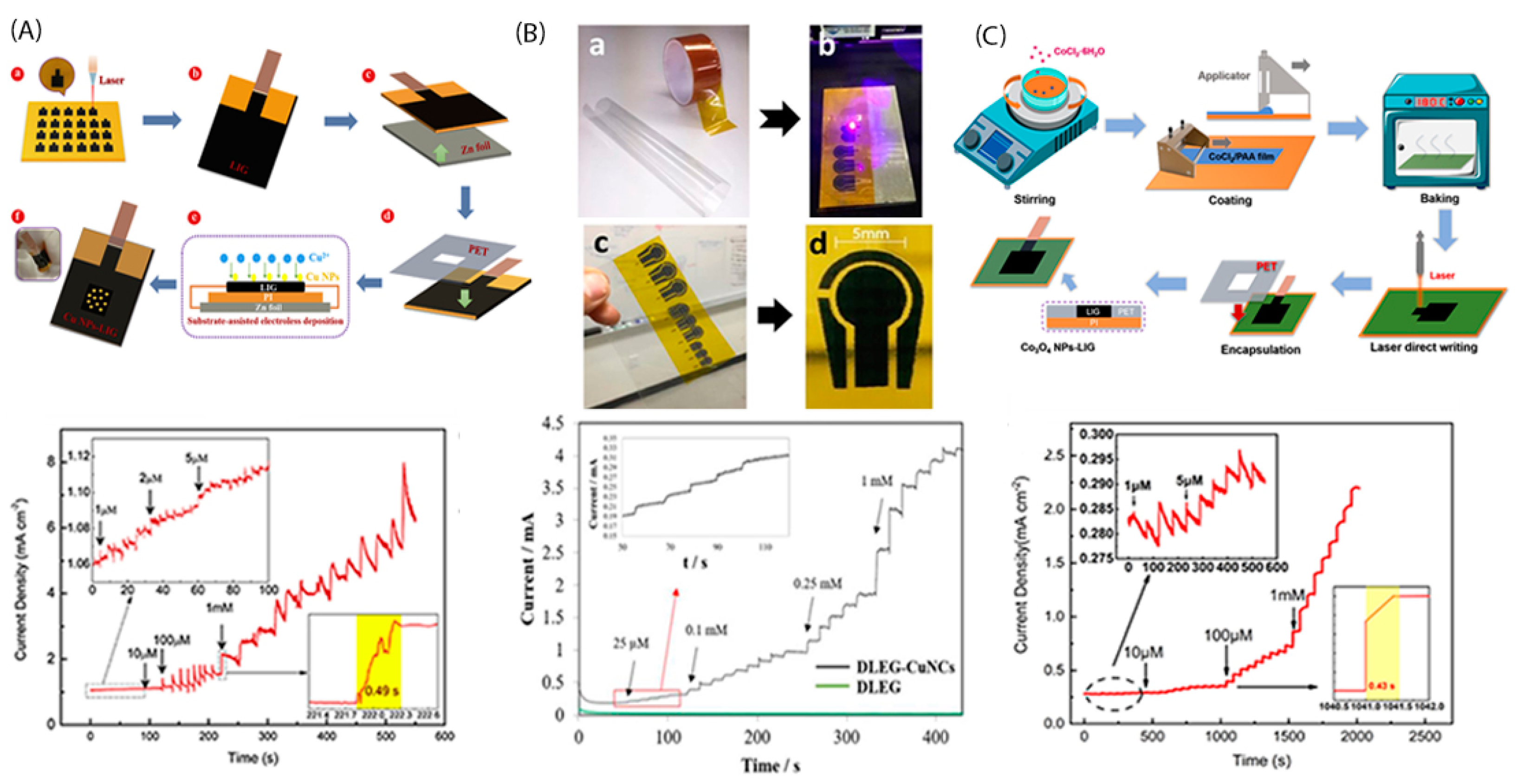
Figure 3. (
A) Cu NPs-LIG synthesis procedure and the respective amperometric response to glucose injection
[28]; (
B) Direct laser engraved graphene (DLEG) decorated with pulse deposited copper nanocubes and the respective amperometric response to glucose injection
[30] (Taping Kapton tape on a thin sheet of PVC (a). Direct laser reduction of the Kapton tape to graphene forming sensor electrodes (b,c). A DREG 3-electrode platform, prepared for further modification (d).) and (
C) LIG assisted encapsulation of Co
3O
4 nanoparticles synthesis method and the respective amperometric response to glucose injection
[31].
4. Polymer Functionalized Graphene-Based Electrodes
Graphene functionalization is a strategy that aims at its surface modification for altering graphene’s chemical and physical properties, mainly reducing the cohesive forces that are deployed between the sheets
[26]. This is separated into two main categories, non-covalent and covalent functionalization
[27]; meaning that in the first case covalent bonds are created, while in the second one the bonds are related to Van der Waals forces.
Graphene as material displays aggregations through Van der Waal interactions, and when defects and functionalities (such as oxygen groups) are in excess, its conductivity and consequently electrocatalytic activity of the graphene-based catalyst, are negatively affected. Therefore, for eliminating such phenomena, organic conducting polymers (with very good electrical conductivity) are used as graphene modifiers and via non-covalent bonding they stabilize graphene’s structure.
Figure 4A shows the structure of a poly-(dimethyl diallyl ammonium chloride (PDDA) functionalized graphene with CuO nanoparticles and the respective amperometric response, to glucose detection. According to catalyst synthesis process the poly-(dimethyl diallyl ammonium chloride (PDDA) polymer, was firstly homogenously dispersed into the graphite oxide solution and then followed the addition of the reducing agent which led to the reduction of graphite oxide to graphene. Its high sensitivity (4982.2 μAmM
−1cm
−2) and the low detection limit (0.20 μM) were attributed to synergistic effects as well as to the large area that PDDA-graphene offered to metal oxides
[32]. Another noteworthy asset of this kind of electrode was its successful testing under human serum without the need of any pretreatment step.
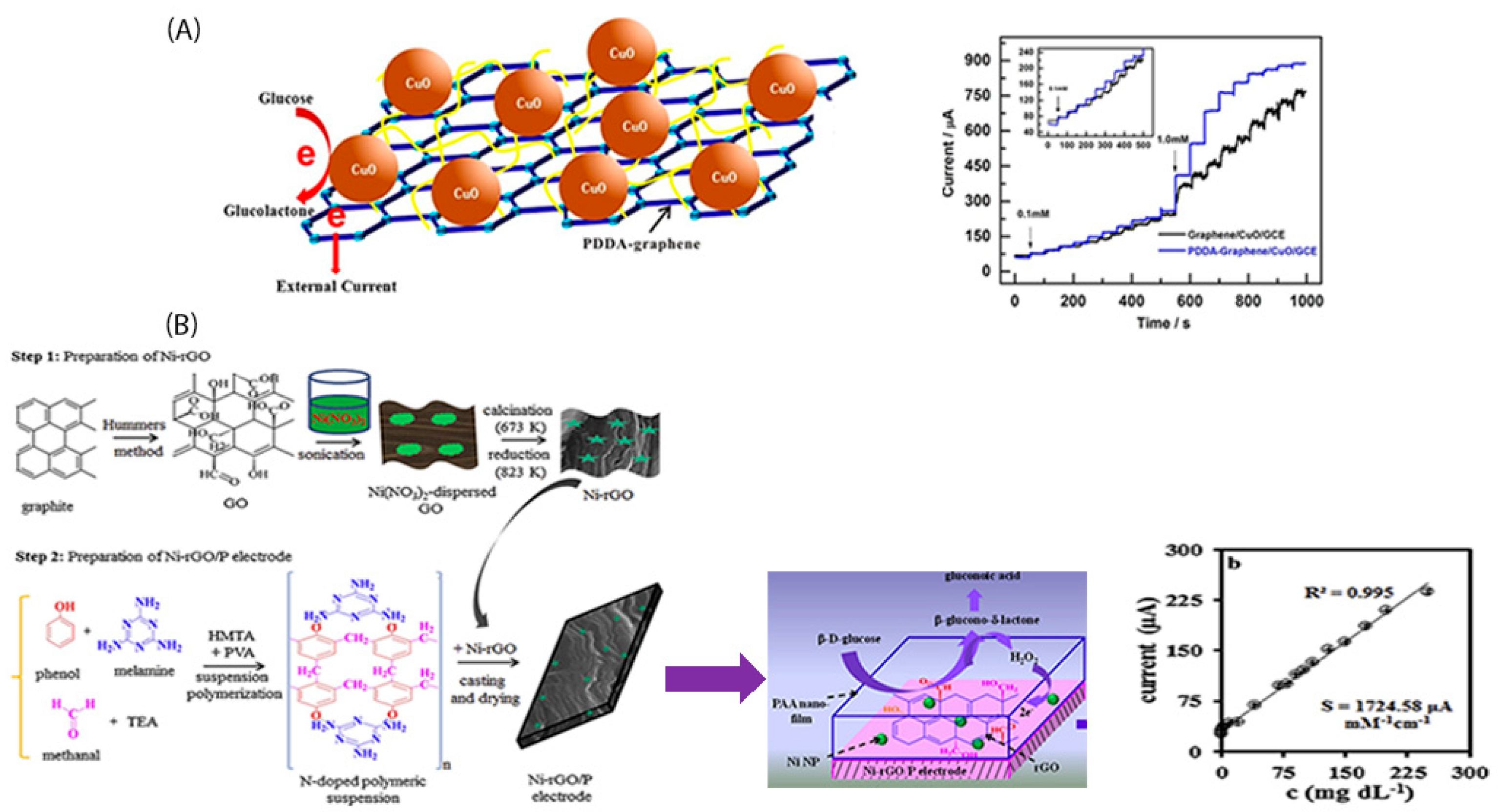
Figure 4. (
A) Poly-(dimethyl diallyl ammonium chloride (PDDA) functionalized graphene decorated with CuO nanoparticles and its respective amperometric response to glucose injection
[32]; and (
B) PAA covered Ni-rGO synthesis procedure (left) and its respective amperometric response to glucose injection
[33].
5. Conclusions
Graphene (or derivatives) structure offer conduction channels improving GER kinetics. More precisely graphene (or its derivatives) significantly reduces electrode’s internal resistance enabling GER via the formation of redox pair peaks, corresponding to the M(II)/M(III) or (M(III)/M(IV)) couple formation. As the resistance is low the charge transfer becomes easier and the metal oxide couples that are responsible for GER progress, are created faster. These faster processes lead to higher stability, sensitivity as well as quicker response of glucose electrochemical sensor operation. For faster and continuous metal couples formation and so higher sensitivity four synthesis methods are mainly recognized among literature works: (i) direct growth of graphene (or oxides) on metallic substrates, (ii) in-situ growth of metallic nanoparticles into graphene (or oxides) matrix, (iii) laser-induced graphene electrodes and (iv) polymer functionalized graphene (or oxides) electrodes. Comparatively, the direct growth of graphene onto metallic substrates and the laser induced graphene electrodes synthesis methods, are more mature. Those two methods use fewer chemical reagents and present higher reproducibility. Moreover, the electrode that presented the highest sensitivity to glucose detection was prepared via the direct growth of graphene onto metallic substrate.