Cancer-associated fibroblasts (CAFs) are key components of the pancreatic tumor microenvironment, maintaining the extracellular matrix, while also being involved in intricate crosstalk with cancer cells and infiltrating immunocytes. Therefore, they are potential targets for developing therapeutic strategies against pancreatic ductal adenocarcinoma (PDAC). However, studies have demonstrated significant heterogeneity in CAFs with respect to their origins, spatial distribution, and functional phenotypes within the PDAC tumor microenvironment. Therefore, it is imperative to understand and delineate this heterogeneity prior to targeting CAFs for PDAC therapy.
1. Introduction
Pancreatic cancer is a lethal malignancy. Five-year survival of patients with pancreatic cancer has only recently reached double digits (~10%)
[1][2], with pancreatic cancer projected to become the second leading cause of cancer-related deaths in the United States by 2030
[3]. About 85% of all pancreatic cancer cases are characterized as pancreatic ductal adenocarcinoma (PDAC)
[4][5]. Surgery remains the only curative option for potentially resectable patients, while patients with more advanced disease are usually treated with systemic therapies, such as chemotherapy
[6][7]. Although the standard-of-care chemotherapy regimens do provide survival benefits, the overall survival still remains dismal. Aggressive biology and resistance to therapy are some of the factors that contribute to these poor outcomes
[6]. Recent studies have demonstrated that, apart from the cancerous epithelial compartment, other cellular and non-cellular elements present in the tumor microenvironment (TME) of PDAC also contribute substantially to its aggressive biology, progression, and metastasis
[8][9]. Therefore, PDAC TME has been extensively investigated as a target to modulate PDAC progression, and to enhance the efficacy of currently available anti-cancer therapies.
1.1 Cancer-Associated Fibroblasts (CAFs) - Origin and Heterogeneity
CAFs are the major non-neoplastic component of the tumor microenvironment in PDAC. As consensus surface markers to distinguish CAFs are still lacking, they are primarily defined as cells that lack lineage markers for epithelial cells (CD326), endothelial cells (CD31), and leukocytes (CD45); possess elongated, spindle-shaped morphology and lack the mutations found within the cancer cells
[10]. CAFs were traditionally thought to originate from Pancreatic Stellate Cells (PSCs), the resident fibroblasts of pancreas
[11]. However, recent studies seem to indicate that PSCs give rise to only a small proportion of PDAC CAFs
[12], and researchers have further identified fibroblast populations expressing PSC-exclusive genes such as
Gli1 and
Hoxb6 which can give rise to PDAC CAFs
[13]. There is also evidence stemming from pancreatic cancer cell-implantation experiments that seems to support a contribution from cells, such as mesenchymal stem cells
[14][15][16] to the CAF pool in pancreatic cancer, although definitive evidence using lineage-tracing studies is lacking in this regard.
Along with a varied origin, CAFs possess significant spatial and functional heterogeneity in the PDAC TME. Multiple studies seem to converge on a two distinct transcriptional programs in the PDAC CAFs: a transforming growth factor-β (TGF-β) driven myofibroblastic program resulting in myofibroblastic CAFs (myCAFs) and an IL-1 driven secretory program resulting in inflammatory CAFs (iCAFs)
[17]. Single cell sequencing studies have also revealed another subtype of CAFs capable of antigen presentation, thus named apCAFs
[18]. These subtypes can be confined to distinct spatial compartments, for eg. myCAFs are found in close proximity to cancer cells while iCAFs are more distant. As more and more evidence come to light, it has become increasingly apparent that the scope of CAF diversity is much greater than previously appreciated, and this calls for more investigations to understand CAF subpopulations, their functions, and their role in tumor progression
1.2 Functions of CAFs
CAFs mediate a variety of effects in the TME and can promote tumor growth by multiple mechanisms (
Figure 1). They shape the desmoplastic stroma characteristic of PDAC by laying down an ECM rich in collagens (
COL1A1,
COL1A2), glycoproteins, and proteoglycans. extracellular matrix (ECM) acts as a barrier to drug delivery, supports tumor growth by providing biochemical cues, contributes to immunosuppressive TME
[19], and enhances expression of matrix metalloproteinases (MMPs), which facilitate cell invasion and metastasis
[20]. Matrix crosslinking enzymes and ECM remodeling also contribute to the increased stiffness of tumor tissue, which can cause hypoxia and a more aggressive cancer phenotype
[21]. It also restricts drug delivery and triggers pro-survival signaling in cancer cells
[22]. As the cancer metastasizes to secondary sites, CAFs favor the establishment of cancer via production of ECM components, facilitating immune exclusion, and providing survival cues to cancer cells
[23].
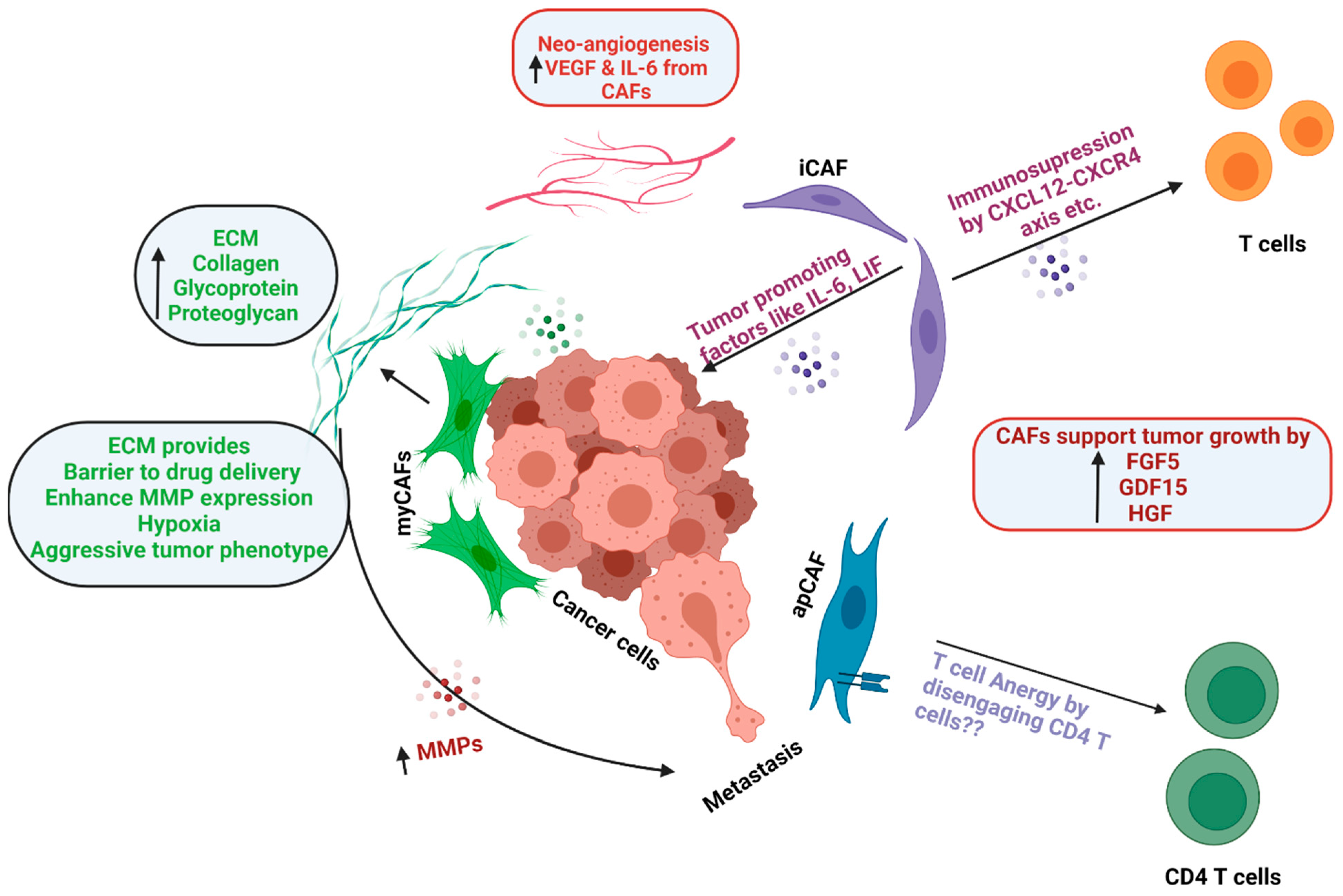
Figure 1. Functions of CAFs. Schematic representation of the functions of CAFs in the tumor microenvironment, such as ECM deposition, immunosuppression, tumor promotion, neoangiogenesis, metastasis, etc.
CAFs further support tumor growth by secreting pro-angiogenic factors such as vascular endothelial growth factor (VEGF)
[24] and IL-6
[25]. They can also provide metabolic support for cancer cells during tumorigenesis. For example, studies have demonstrated that CAFs can provide amino acids for cancer metabolism through processes such as macropinocytosis
[26] and autophagy
[27].
CAFs secrete a battery of growth factors and cytokines that can promote tumor growth and modulate the response to therapy. CAFs have been reported to establish an immunosuppressive environment by secreting biomolecules, such as IL-6, CXCL12, TGF-β, growth arrest-specific protein 6 (GAS6), fibroblast growth factor 5 (FGF5), growth differentiation factor 15 (GDF15), and hepatocyte growth factor (HGF), which promotes invasive and proliferative behavior in cancer cells
[28][29][30]. CAFs also have immunosuppressive effects on immune cells, such as CD8+ T cells, regulatory T cells (Tregs), and macrophages
[19] caused via IL-6, CXC chemokine ligand 9 (CXCL9), and TGFβ
[31]. CAFs also prevent CD8 T-cell infiltration in tumors
[32], and they recruit immunosuppressive cell populations, such as myeloid-derived suppressor cells (MDSCs) and neutrophils
[19].
2. CAFs as Therapeutic Targets
The critical and diverse functions performed by CAFs in the tumor microenvironment make them attractive targets for antitumor therapies. Modulation of the tumor microenvironment through direct targeting/depletion of CAFs, or disrupting their cross-talk with cancer cells and immune cells, has been attempted with varying degrees of success in both preclinical studies and clinical trials (Table 1). These studies have also provided insights into mechanisms by which CAFs promote tumor growth.
Table 1. Clinical trials targeting CAFs in PDAC.
S. No. |
Target |
Name of Therapeutic |
Rationale Based on Pre-Clinical Studies |
Current Status |
ClinicalTrials.gov Identifier |
1. |
Hedgehog Pathway |
IPI-926 |
Inhibition of the Hedgehog Pathway, leading to reduced CAF activation |
Phase II was halted due to the early detection of a shorter median overall survival (OS) in the experimental arm, compared to the placebo arm. |
NCT01130142 |
2. |
Hedgehog Pathway |
Vismodegib |
The phase Ib/II randomized clinical trial, evaluating the addition of Vismodegib to gemcitabine, showed no treatment benefit for OS or progression free survival (PFS). |
NCT01195415 |
3. |
Hyaluronic acid |
PEGPH20 |
Depletion of stroma by PEGPH20, which may synergize with immunotherapy |
Clinical trials failed to show any benefit. |
NCT03634332 |
4. |
Angiotensin receptor |
Losartan |
Attenuation of collagen and hyaluronan deposition by CAFs through inhibition of TGF-β signaling |
Encouraging results in locally advanced PDAC |
NCT03563248 |
5. |
Lysyl oxidase like-2 (LOXL2) |
Simtuzumab |
Inhibition of matrix-remodeling enzyme Lysyl oxidase-like 2, an ECM remodeling enzyme |
Study completed, and addition of Simtuzumab to gemcitabine did not improve clinical outcomes |
NCT01472198 |
6. |
CXCL12-CXCR4 Axis |
Olaptesed (NOX-A12) |
Modulation of PDAC TME by reducing immunosuppressive factors, as CXCL12 secreted by iCAFs promotes tumorigenesis by reducing CD8 T cell infiltration |
Clinical trial ongoing |
NCT03168139 |
7. |
CXCL12-CXCR4 Axis |
BL-8040 CXCR4 Antagonist |
Clinical trial ongoing |
NCT02826486 |
8. |
IL-6 |
Siltuximab |
Combination of IL-6 and PD-L1 blockade decreases tumor growth, improves survival, and leads to increased infiltration of effector CD8+ T cells |
Clinical trial ongoing |
NCT04191421 |
9. |
Vitamin D receptor (VDR) |
Paricalcitol (Vitamin D Receptor Agonist) |
Modulating signaling in tumor microenvironment. CAFs highly express VDR, and treating them with Vitamin D can induce a quiescent phenotype |
Clinical trials ongoing |
NCT03520790 |
NCT03300921 |
NCT02754726 |
10. |
Vitamin D receptor (VDR) |
Vitamin D3 |
Clinical trials ongoing |
NCT03472833 |
11. |
Stroma |
All Trans Retinoic Acid (ATRA) |
Inducing CAF quiescence and decreasing motility, leading to decreased tumor growth through decreased Wnt-β Catenin signaling |
Clinical trials ongoing |
NCT03307148 |
12. |
IL-1R |
Anakinra (IL-1R antagonist) |
By switching iCAF to a myCAF phenotype |
Clinical trials ongoing |
NCT02550327 |
CXC chemokine ligand 12 (CXCL12).
3. Conclusions
The PDAC stroma is a complex and dynamic compendium of cellular and biochemical components, and plays a significant role in tumor progression and metastasis. The stromal compartment has undergone a great deal of investigation in the past decade, thanks to single-cell RNA-sequencing techniques, and as a result, we now understand that CAFs are heterogenous entities, and this has changed the rationale of targeting CAFs in PDAC therapeutics. Now, we are well aware that some CAFs can behave as protumorigenic, while others as antitumorigenic, and therefore, their targeting in solid malignancies demands their thorough characterization. It will be important to delineate whether this heterogeneity results from variable origins of CAFs, or as a consequence of variations in autocrine and paracrine signals in the microenvironment. Current reports suggest that iCAF and apCAF are attractive targets that can be reprogrammed to transform into quiescent CAFs. Still more work is required in terms of their lineage determination and extraction of a good number of viable CAFs from dense ECM, so that their characterization also improves. Moreover, we need to evaluate how durable these subtypes remain under therapeutic pressures, and what their contribution is to therapy resistance in PDAC.
Although there is no approved stroma-targeting therapy yet, a number of clinical trials are underway. Failures of some trials, such as targeting the Hedgehog pathway, have been disappointing, but helped in expanding our horizon of CAF biology. Now, as we understand the CAFs better than ever, it is important to design the clinical trials targeting CAFs carefully, so that CAF-targeting agents with currently available therapeutics will lead to better outcome in PDAC patients.
This entry is adapted from the peer-reviewed paper 10.3390/ijms222413408