Commensal, anti-inflammatory gut microbiota metabolises complex carbohydrates into short-chain fatty acids (SCFA). SCFA fulfil various roles, including activating and inhibiting the inflammatory response and different metabolic pathways
[79]. SCFA levels vary between obese and lean individuals
[80]. Butyrate, a type of SCFA, contributes to the prevention of metabolic disorders
[81] and helps retain the IB’s integrity, thus reducing the rate of LPS translocation through the intestinal epithelium
[81]. SCFA can also prevent HFD-induced obesity in mice by altering gut microbiota
[82]. Furthermore, butyrate stimulates nuclear transcription factor-peroxisome proliferator-activated receptor gamma (PPAR-γ), which in turn inhibits the pro-inflammatory NF-κB pathway
[83][84]. It also inhibits interferon-gamma (IFN-γ) signalling to suppress inflammation
[85].
2. Interactions between the Gut and Distant Organs
Gut microbiota communicates with the brain through several communication channels known as the microbiota–gut–brain axis. The communication routes include tryptophan metabolism, the vagus nerve, microbial metabolites, and the immune system
[86]. Even though the notion of a gut–brain axis is relatively new, the evidence is exponentially rising
[87][86][88][89][90][91][92].
Interaction between microbiota and the host’s tissues within the IB can result in the secretion of chemokines, cytokines, neurotransmitters, endocrine messengers, neuropeptides, and microbial by-products such as LPS
[86]. These molecules can then penetrate the vascular and lymphatic systems, impact neural messages carried out by vagal and spinal afferent neurons, communicate with the brain regarding the host’s health status and influence behaviour
[86]. Most of the host–microbiota interactions occur within the IB, where the exchange of molecules mediates communication between the gut and immune system
[93]. The intestinal epithelium also houses enterocytes, secretory cells, chemosensory cells and gut-associated lymphoid tissue, participating in the immune response
[94].
The enterocytes play a vital role in the innate immune system response by releasing pro- and anti-inflammatory cytokines and chemokines, whereas B lymphocytes in Peyer’s patches produce immunoglobulins
[95]. Intestinal epithelial pattern recognition receptors (PRRs) identify molecular patterns unique to the specific microorganisms
[96][97]. These include Toll-like receptor (TLR) family members, which mediate pro-inflammatory responses
[98]. In addition, some metabolites of commensal bacteria (such as LPS) can activate the innate immune system and, thus, modulate neural responses
[86][99].
The integrity of the blood–brain barrier (BBB) can be affected by systemic pro-inflammatory signalling induced by a HFD
[100][101][102], leading to brain inflammation and injury. This may not be linked to obesity, as transplantation of microbiota from mice fed a HFD caused significant disruptions in exploratory, cognitive, and stereotypical behaviour in non-obese recipient mice fed a standard diet
[103]. This study provided direct evidence that HFD-induced changes to the gut microbiome are sufficient to disrupt brain function in the absence of obesity. Furthermore, previous studies have shown that germ-free mice exhibit increased BBB permeability, with downregulation of TJ proteins claudin-5 and occludin. Nevertheless, exposure of germ-free mice to specific-pathogen-free mice followed a reduction in BBB permeability and upregulation of TJ proteins
[104].
The gut microbiota can also modulate the host’s behaviour and brain function
[89][105][106][107][108]. Of interest, germ-free mice displayed cognitive deficits, similar to mice infected with
Citrobacter rodentium and exposed to acute stress
[109][110]. Several studies have reported gut microbiota as a critical player in neuroinflammation, neurodegeneration and mental illness development
[111][112]. Gut dysbiosis has been said to play a role in the aetiology of neurodegenerative diseases such as Alzheimer’s disease (AD)
[113], Parkinson’s disease (PD)
[114], multiple sclerosis
[115] and amyotrophic lateral sclerosis
[116]. In AD, an imbalance in the microbiome is associated with the co-localisation of LPS with amyloid β-protein (Aβ) 1–40/42 in amyloid plaques and around blood vessels
[117]. LPS binds to microglial receptors (TLR2, TLR4 and CD14), activating the NF-κB (p50/p60) complex, which initiates neuroinflammatory processes
[118][119].
Regarding PD, bowel inflammation can provoke the progression of the disease
[120]. Colonic hyperpermeability in PD patients has been linked with increased α-synuclein and
E. coli accumulation in the sigmoid colon
[121]. The microbiome landscape in PD is significantly shifted, with decreased levels of bacteria that produce anti-inflammatory mediator butyrate (
Blautia,
Coprococcus, and
Roseburia) and increased levels of LPS-producing bacteria (
Oscillospira and
Bacteroides)
[114]. Furthermore, specific changes in the gut microbiome have been linked with the severity of symptoms and elevated serum cytokine levels seen in PD
[122]. This further supports a link between changes in gut microbiota, systemic inflammation and PD.
IBD has also been linked with a higher incidence of neurodegenerative disorders, including dementia
[123][124]; however, the mechanisms are not well understood.
In summary, factors such as a HFD can cause gut dysbiosis, hyperpermeability of the IB and infiltration of pathogens and microbial solutes into the circulation, thus contributing to chronic systemic inflammation
[125]. Chronic inflammation can disrupt the BBB’s integrity, facilitating the infiltration of pathogens and pro-inflammatory cytokines into the brain, resulting in neuroinflammation and neurodegeneration
[126][127][128][129] (
Figure 1 and Figure 2 ).
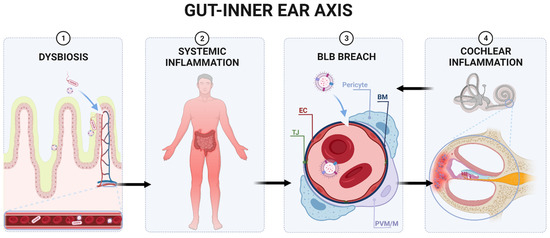
Figure 1. Proposed gut–inner ear axis resulting in sensorineural hearing loss (SNHL). (1) Gut dysbiosis induced by a high-fat diet can damage the intestinal barrier and cause a leaky gut. (2) This allows gut microbiota and bacterial toxins such as lipopolysaccharide to infiltrate the bloodstream and cause a systemic inflammatory response. (3) Pathogens and inflammatory cytokines reaching the inner ear can damage the blood–labyrinth barrier (BLB). (4) Infiltration of pathogens to the inner ear leads to the activation of resident macrophages, the release of pro-inflammatory cytokines, and the overproduction of reactive oxygen species (ROS), causing apoptosis of damaged cells and immune cell infiltration in the lateral wall of the cochlea (spiral ligament and stria vascularis). Inflammatory processes in the cochlea might further increase the BLB’s permeability, perpetuating inflammation. Unresolved inflammation leads to damage of sensorineural structures, eventually causing SNHL. Abbreviations: EC, endothelial cell; TJ, tight junction; BM, basement membrane; PVM/M, perivascular-resident macrophage-like melanocytes.
Figure 2. Flowchart showing the potential link between a high-fat diet and sensorineural hearing loss (SNHL) based on current literature.