Improvements in bioengineering methodology and tools have allowed for significant progress in the development of therapeutics and diagnostics in medicine, as well as progress in many other industries, such as materials manufacturing, food and agriculture, and consumer goods. Glioblastomas present significant challenges to adequate treatment, in part due to their immune-evasive nature. Rational-design bioengineering using novel scaffolds, bio-based materials, and inspiration across disciplines can push the boundaries in treatment development to create effective therapeutics for glioblastomas.
1. Introduction
Glioblastoma (GBM) has the most devastating prognosis of all primary brain tumors. Despite decades of research and therapeutic innovation, the median survival for GBM patients remains at only 15–16 months, even for patients receiving optimal multimodal therapy
[1]. GBM has unique characteristics that make treating the disease and eradicating tumors particularly challenging. All tumors recur because the highly migratory and infiltrative nature of GBM renders surgical resections incomplete
[2]. Additionally, because these tumors are largely located in the brain, any therapeutics given to patients must cross the blood–brain barrier (BBB), which blocks at least 98% of both small- and large-molecule drugs
[3][4]. Moreover, once drugs cross the BBB, they must do so in quantities effective for treatment, which is another hurdle to overcome
[3]. While the BBB is traditionally difficult to overcome, however, nanotechnologies present opportunities for innovation to help drug molecules access brain tissue
[5].
GBM are “cold” solid tumors, which resist host immune responses by suppressing natural killer cells and inducing T-cell apoptosis
[6][7]. They are also classified by high intratumoral clonal neoantigen heterogeneity, which presents a two-way challenge: drugs must target enough clonal neoantigens to be effective immunotherapeutics, but drugs that target a large amount of neoantigens risk also targeting healthy tissues
[6][8]. Clonal neoantigen heterogeneity also contributes to T-cell exhaustion, a phenomenon that presents in several viral infections and tumor types but most severely in GBM
[9]. T-cell exhaustion is most prevalent among tumor-infiltrating lymphocytes and prevents them from targeting GBM tumor cells, leading to high levels of T-cell dysfunction observed in murine GBM models as well as human patient-derived GBM cell lines
[9]. GBM is additionally known for its plasticity, especially of druggable targets
[6][10]. Furthermore, glioma-associated macrophages and microglia (GAMs) exhibit intratumoral spatial heterogeneity of inflammatory phenotypes; core GAMs have pro-inflammatory signatures while peripheral GAMs have anti-inflammatory signatures in GBM tumors
[11][12]. These regional and spatial differences allow for GAMs to play important roles in tumor growth and evolution, including their function of inducing GBM cell migration and invasion
[11][12]. Together, these factors present uniquely challenging difficulties in targeting GBM tumors for complete eradication and in developing successful treatments.
Immunotherapies and bioengineered medicines have emerged as a promising new approach to treating aggressive tumors and have undergone accelerated development in the past ten years. Novel therapeutic avenues include the development of immune checkpoint blockade therapeutics, chimeric antigen receptor T cells (CAR T cells), dendritic cell (DC) vaccines, and engineered nanomedicines. Checkpoint blockade therapies have drastically improved patient outcomes for melanoma
[13] and non-small cell lung cancer
[14]; engineered T cells have done the same for hematologic cancers
[15][16] and are FDA-approved treatment modalities for those diseases
[17]. Unfortunately, they have not been as effective for GBM. Anti-PD1 therapy, which targets a popular immune checkpoint, has not been effective as a monotherapy in GBM, and efficacy of combination checkpoint blockade treatment in GBM patients is not fully established
[18][19]. Median patient survival from three different CAR T-cell trials for GBM has been less than one year, though a select few patients have had extended survival beyond two years
[19]. The main barriers to success for these therapies include the challenging GBM factors mentioned previously, such as intratumoral clonal neoantigen heterogeneity and plasticity, as well as poor T-cell infiltration into the tumor microenvironment
[6][19]. An exciting new category of immunotherapy candidates for GBM worth noting is dendritic cell vaccines: preliminary data from the Phase 3 trial for DCVax
®-L may show increased survival for patients with newly diagnosed GBM
[2]. However, this study is still ongoing, and these results will need to be replicated before full approval for standard of care. Additionally, artificial antigen presenting cells (aAPCs) engineered to target cancer-specific ligands have shown promise in efficacy and manufacturability
[20][21].
While efforts to identify higher quality neoantigens and target mechanisms of resistance hold promise, progress in patient outcomes has been slow. To aid in these efforts, bioengineering and the rational design of novel therapeutics have been and should continue to be applied to allow for more dynamic iteration of candidate drugs to better counter the challenges that GBM presents for effective treatment, summarized in
Table 1. Protein engineering and optimization can help therapeutics better overcome the blood–brain barrier, extend their half-life and stability in various environments, and add useful functionalities to agents that assist in tumor killing. Additionally, the use of engineered biomaterials and nanotechnology can improve the delivery of therapeutics and tumoral access, especially to and in the brain. Bioengineering has allowed for significant innovation thus far, both in the research pipeline through the development of novel immunotherapeutics against GBM and in reaching GBM patients in ongoing and future clinical trials
[22].
Table 1. Summary table of opportunities and challenges for bioengineered therapies in GBM. Images created with Biorender.com.
|
Examples Discussed |
Advantages |
Challenges |
Antibodies 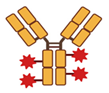 |
|
|
|
Alternative Scaffolds 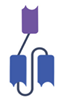 |
-
Anticalins, Affibodies, DARPins, Monobodies, etc
|
-
High stability and long half-life in vivo
-
Can be engineered with multi specificity to tackle GBM heterogeneity
-
Added functionalities beyond Abs, such as linkers
-
Easy and cost-effective manufacturing
|
-
No clinical trials currently in GBM
-
Concern for immunogenicity in vivo
-
Early stage of investigation, much more to learn in terms of safety and efficacy
|
Engineered Cells & Particles 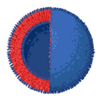 |
|
-
Can modify cells to express virtually any ligand
-
Stable in vivo
-
Long history of investigation for many applications, including GBM
|
-
Still rely on trafficking of cells to tumor site, known to be a challenge in GBM
-
CAR T cells have been known to cause cytokine storm and other off-target adverse effects
|
Polymeric Biomaterials 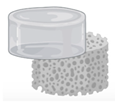 |
|
-
Allows sustained release of particles or engineered cells, therefore single implantation/injection can yield long-term therapy
-
Can be used to trap highly migratory GBM cells and kill them, or trap exhausted/immunosuppressive T cells
-
Long history of use in tissue engineering
|
|
DNA Structures 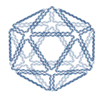 |
|
-
Ideal for drug delivery: self-assembly, complex structure formation, biocompatibility, and degradability
-
Can carry a variety of cargoes such as drugs, siRNA, CpG, proteins & enzymes, and more
|
|
Implantable Nanoreactors 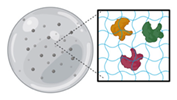 |
|
-
Can provide continuous, robust conversion or synthesis of molecule of interest based on environment
-
No limitations of living cells
-
Can be loaded with multiple enzymes to perform a cascading reaction pathway, or multiple different reactions to target heterogeneity of GBM
-
Stable over months
|
-
Very limited studies exist on cell-free stabilized enzymes as a therapy, while more precedent exists in biomanufacturing
-
Safety profile in vivo is a possible concern with a continuous reaction system
|
2. Discussion
Bioengineered therapeutics have demonstrated advanced functionalities to improve patient outcomes in clinical trials and laboratory disease models. While such engineered therapeutics specifically designed for immunotherapy are few, they represent a growing opportunity for rational design in treating currently uncurable diseases, such as GBM (Table 1).
Crucial to the viability of any immunotherapeutic is biocompatibility and lack of general immunogenicity once administered, such that an adverse immune storm is triggered. As we have discussed, advances in bioengineering have allowed for new drugs with a tolerable safety profile when administered, opening the door to use these novel structures and backbones in the design of anti-GBM therapeutics that accel where currently available treatments fall short. Biomaterials, such as hydrogel “vaccines” and CART-mimicking nanovesicles loaded with sustained-release drug, that mimic intended actions of existing immunotherapuetics that have been slow to show significant effect in GBM but introduce key innovations allowing for potential success are promising for future therapeutic development.
There remains significant room for creativity in building novel bioengineered therapeutics. For example, cell trapping hydrogels can be employed to lure and activate exhausted T cells, which in GBM are thought to contribute greatly to immunosuppression and poor outcomes. Additionally, implantable nanoreactors of stabilized enzymes are an exciting frontier that provide long-term high throughput therapy of any kind. Future nanoreactors could be designed to breakdown immunosuppressive factors in circulation or locally produce active tumor-killing compounds by conversion of circulating factors either produced in the tumor microenvironment inherently or injected as part of the system design.
The bioengineering revolution has opened new possibilities in medicine, agriculture, food, and materials manufacturing that are only limited by our imagination. For immunotherapy in GBM, bioengineering has allowed for leaps of progress towards building smarter, safer medicines that have the potential to improve quality of life and patient prognosis. As we continue innovating in this field, cross-discipline collaboration between immunology, oncology, neurosurgery, infectious disease, engineering, etc. will be just as important as collaboration cross-industry, as synthetic biology has become popularized for its application across sectors. We can also apply some of what we learn and publish from engineering immunotherapies against brain cancer to biomanufacturing of all goods for human prosperity through the unifying power of bioengineering.
This entry is adapted from the peer-reviewed paper 10.3390/immuno2010004