Dioxins and related compounds are environmental xenobiotics that are dangerous to human life, due to the accumulation and persistence in the environment and in the food chain. Cancer, reproductive and developmental issues, and damage to the immune system and endocrine system are only a few examples of the impact of such substances in everyday life.
1. Introduction
Dioxins are among the most toxic and persistent organic pollutants (POPs). They are principally released into the atmosphere as undesired products of various combustion and industrial processes
[1] such as incineration of municipal and medical solid waste, backyard waste burning, paper and wood pulp chlorine bleaching, coal fired power plants, and also from natural processes like forest fires. Furthermore, these pollutants also occur as contaminants in several pesticides, herbicides, and fungicides
[2].
When accidently released in the various environmental matrices, like soil and water, dioxins accumulate in plants and animal tissues, until they reach human tissues where accumulate at higher and higher concentrations, mainly in the fatty tissues, through food chain biomagnifications processes.
These halogenated organic POPs mainly belong to three families of molecules, i.e., polychlorinated dibenzo-p-dioxins (PCDDs), polychlorinated dibenzofurans (PCDFs) and polychlorinated biphenyls (PCBs); their molecular structures are presented in Figure 1.
Figure 1. General molecular structures of chlorinated dioxins (PCDDs), furans (PCDFs) and biphenyls (PCBs).
All these compounds together with Polychlorinated Diphenyl Ethers (PCDEs) belong to the main family of Polyhalogenated Aromatic Compounds (PHAs). PCBs and PCDEs are industrial compounds or by-products, mainly present as impurities in chlorophenol preparations. These two classes of POPs have been detected in the environment and especially in chemical-waste dumpsites. PCBs, in particular, are synthetic PHAs used as industrial reagents in the past. Because they persist for long times in both the environmental matrices and in tissues of living organisms, their production and utilization were discontinued.
PCDDs and PCDFs have two benzene rings connected by one (furans) or two (dioxins) oxygen atoms. The two benzene rings can bind from one to eight chlorine atoms, generating a wide family of congeners (75 PCDD congeners and 135 PCDF congeners) whose molecular reactivity toward cellular targets can change dramatically, determining different levels of toxicity. Within these 210 congeners, the 2,3,7,8-tetrachlorinated dibenzo dioxins (TCDD) species have been classified as the most toxic. Toxicity drastically decreases when non-lateral chlorines are present or when lateral chlorines are removed from the two aromatic rings
[3]. Laterally chlorinated dioxins and especially TCDD show the highest affinity toward the AhR (Aryl hydrocarbon Receptor) receptor, a signal transducer protein which is responsible of the biological effects cascade following the xenobiotic–receptor interaction
[4][5][6]. There are seven laterally chlorinated PCDDs and ten laterally chlorinated PCDFs. The toxicity equivalent factor (TEF)
[7] expresses the toxicity of dioxins, furans and PCBs in terms of the most toxic congeners, i.e., the 2,3,7,8-TCDD and 1,2,3,7,8-pentachlorodibenzo-p-dioxin, whose TEF value has been set to 1. It must be taken into account that dioxins are extremely toxic molecules; indeed, they can cause cancer, reproductive and developmental aberrations, immune system damages, and can deeply interfere with the endocrine system
[8][9][10][11].
2. Optical Monitoring of Dioxins and Related Compounds, a Screening of Old and New Mid-IR Light Sources and Techniques
Optical monitoring offers many advantages with respect to the cited techniques like chromatography or high-resolution mass spectrometry in terms of costs, destructive sampling and fast detection. Optical techniques can be divided into two main classes: (1) those which employ wide wavelength range sources and (2) those which use narrow wavelength range sources. Let us discuss them separately.
2.1. Wide Wavelength Range Sources
The use of Mid-IR radiation to detect PCDD/Fs and related compounds allows discriminating between congeners, since their own fingerprints in this spectral region characterize these molecules.
The most frequent use of the absorption spectroscopy in Mid-IR lies in the identification of substances through their characteristic molecular vibrations. Furans and dioxins, and in general, molecules with a similar structure, are not in gaseous phase at room temperature, and high temperatures (more than 550 °C) are needed to perform gaseous spectroscopy. However, in order to work at room temperature, it is possible to carry out spectroscopy in liquid phase, dissolving the target molecules in a Mid-IR transparent solution
[12][13]. In both gaseous and liquid spectroscopy, the detected absorption peaks are tens of cm
−1 wide. In order to detect these bands, techniques and components spanning a wide frequency range are needed. In this framework, a consolidated technique is Fourier-Transform Infrared Spectroscopy (FT-IR)
[14]. In this scheme, sketched in
Figure 2, using a broadband source, typically a lamp, an interferometric pattern is recorded from the light interacting with the sample. By using the Fourier Transform approach, it is possible to reconstruct the absorption spectrum of the substance under analysis.
Figure 2. Principles of the Fourier-Transform Infrared Spectroscopy (FT-IR).
The resolution of commercial instruments is better than 1 cm
−1. This technique has been used for measuring dioxins, furans and related chlorinated organic molecules. In
Figure 3 and
Figure 4, the infrared spectra of 13 of the 17 most toxic congeners of dioxins dissolved in carbon tetrachloride (CCl4) was reported
[13].
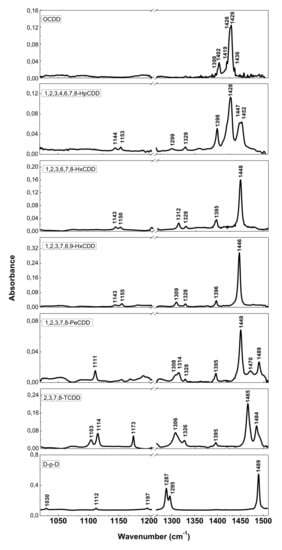
Figure 3. FT-IR spectra of PCDDs and dibenzo-p-dioxin (D-p-D). Reprinted with permission from
[13], copyright 2014.
Figure 4. FT-IR spectra of PCDFs and dibenzofuran (DBF). Reprinted with permission from
[13], copyright 2014.
2.2. Narrow Wavelength Range Sources
Mid-IR Laser technology is nowadays gaining interest due to new device development. However, sometimes chemical analysis and biological assays need a fast response, not necessarily quantitative but at least indicative of the presence of a toxic molecule. Therefore, a class of sensors characterized by cost-effectiveness, ready for real-time and on-site analysis is highly desirable. In many cases, sensors might be used for the determination of dioxins and dioxin like-PCBs throughout the environment and the food chain, involving various types of specimens such as water, air, soil, food, animal tissues and so on. So far, we compared different techniques based on wide spectrum sources. However, there are other classes of optical sensors based on lasers with limited tunability (a few nm at most) and possibly cheaper. One of them takes advantage of plasmonic resonances, and is able to detect complex organic molecules
[15][16][17][18][19][20]. These sensors would deserve a broader discussion, so we refer also to other review articles and books present in the literature
[15][21].
Basically, the interaction between the target molecule and the structure (nanoparticles, nanomaterial, fiber sensors), provided with plasmonic resonances, produces a relevant effect in absorption, fluorescence enhancement, or frequency shifting of a laser beam. In the latter case, when the molecule is on the plasmonic structure, the change of physical conditions at the interface produces some changes in the plasmon coupling, namely, an easily detectable resonance shift. In this way it is possible to obtain a very sensitive sensor, tailored onto a specific target molecule or molecular class. In most cases, the plasmonic sensor needs to be functionalized to enable the sticking of the molecule to the structure, so making the detection possible. A scheme of a kind of plasmonic sensor is reported in Figure 5.
Figure 5. Sketch of a plasmonic sensor. In this particular case the light is used to “read” the sensor. Molecule “detection” is performed by means of the interaction between the sample and a material supporting the surface plasmons.
In the literature there are some examples of plasmonic sensors for measuring dioxins, dioxin precursors, polychlorinated biphenyls and atrazine
[22][23]. The strong point of these sensors is the very high selectivity and sensitivity
[23], down to concentrations of the order of ng/mL. In some cases, single molecule detection could be achieved. It is fundamental specifying that this kind of plasmonic sensor detects the molecule sticking on the surface, so the chemical selectivity is due essentially to the selectivity of the functionalized material. For example, if this material is able to capture all the molecules of dioxin and furan families, the sensor could detect also a single molecule but it would not discriminate between the most toxic 2,3,7,8 TCDD and the non-toxic DBF. For evident reasons, plasmonic sensors could suffer polluting phenomena, so they may have to be cleaned or replaced. This could not be a real problem, as using some classes of plasmonic structure or functionalizing materials, plasmonic sensors could be very cheap.
Similar mechanisms of operation are common to other kind of sensors based on Surface-Enhanced Raman Scattering (SERS),
[24][25][26][27][28][29], fluorescence quenching and enhancement
[30] and surface photo voltage
[31]. Raman spectroscopy is a well-established technique, based on the inelastic scattering of light. Starting from a single-frequency laser excitation it is possible to get from the target molecule one or more emissions at lower (Stokes) or higher (anti-Stokes) frequencies. The frequency differences, with respect to the pumping laser, are typical of each target, so allowing selectivity. Usually the Raman signal deriving from a single layer of molecules is very low. When plasmon resonance is involved, the effect rises by a factor up to 108, and this allows single molecule detection. For many years, the poor reproducibility of the metal coatings needed for SERS limited the applications of this technique. Nevertheless, since a couple of decades ago, this technological issue has been overcome and applications became possible. In the literature, it is possible to find examples of detection of dioxins
[24] and of other molecules, like pesticides
[32].
SERS is one of the most employed spectroscopic techniques for ultrasensitive detection in chemistry and biology. One of the most important requirements in this field is the design of advanced materials capable of generating high-quality SERS signals. Zhu et al.
[27] used Ag nanoplate-assembled films as SERS substrates for the rapid detection of PCB-77. The authors obtained a detection limit of about 10
−7 M (mol/L) and found a linear dependence between the logarithmic concentrations of PCB-77 and the fingerprint peaks intensities. The authors used the same Ag nanoplate-assembled also to distinguish the characteristic peaks of different PCBs congeners in mixed solutions. Abalde-Cela and co-workers
[24] obtained the SERS spectrum of 2-benzoyldibenzo-p-dioxin at a concentration lower than 10
−8 M (mol/L), a detection level that matches current immunological methods. They used a SERS substrate based on thin films made by exponential layer-by-layer, infiltrated with silver nanoparticles. The authors found a characteristic vibrational pattern characterized by bands at 1392 (ring stretching) and 930 cm
−1 (ring breathing), and smaller intensity bands at 1641 (CO stretching), 1605 and 1445 (ring stretching), 1296 (CO stretching), 852 (CH wagging), and 791 (ring deformation) cm
−1.
These sensors represent a rapid, direct, and ultrasensitive detection method for environmental pollutants from different matrices.
Wang and co-workers
[30] reported on the use of a sensitive and selective fluorescent membrane for rapid detection of trace 2,2′,4,5,5′-pentachlorinated biphenyl (PCB101). The fluorophore phenyl isothiocyanate (PICT) was immobilized onto porous anodic aluminium oxide membrane. The fluorescence of this membrane is enhanced after adding the analyte PCB101 into the membrane, due to the interaction between the fluorophore PITC and the analyte PCB101. The fluorescence intensity increased with the PCB101 concentration in the low range below 1 ppm, and the membrane showed good selectivity.
This entry is adapted from the peer-reviewed paper 10.3390/ijms20112671