1. Introduction
The 14-3-3 (or GF14) proteins belong to the conservative family of proteins, present in all eukaryotes. 14-3-3s modulate activity of various targets in a cell via protein–protein interaction in phosphorylation-dependent mode
[1]. The first protein from the family was discovered in a cow brain in 1967
[2]. 25 years later, 14-3-3s almost simultaneously were found in plants:
Arabidopsis thaliana [3], maize
Zea mays[4], spinach
Spinacea aleracea, pea, and herbaceous plant
Oenothera[5]. In plants, 14-3-3 family members are involved in regulation of processes such as primary metabolism, cell division, growth and differentiation, light, hormone and stress signalling, as well as plant innate immunity
[6][7][8][9] [10]. Notably, the 14-3-3 proteins do not have their own enzymatic activity. Its mechanism of action corresponds with a facility to perform physical protein–protein interactions. Intensive investigations revealed more than 300 potential targets of 14-3-3 proteins
[8]. The 14-3-3 proteins interact with each other and form homo- or hetero-dimers
[11]. Thus, dimers have two domains for interacting with target proteins, which are assumed to be phosphorylated in most cases at the amino acid residues of serine or threonine
[12][13].
2. Diversity of 14-3-3 isoforms in plants
Green plants have the highest number of 14-3-3 isoforms. Protists and fungi have from one to four isoforms
[14]. In mammals, seven 14-3-3 isoforms were discovered and named by Greek letters (beta/alpha, gamma/delta, epsilon, etc.)
[1]. In plants, isoforms were denoted firstly also by Greek letters, but starting from the end of the alphabet: omega, psi, chi, phi, etc. Alternatively, 14-3-3s in plants are known as General Regulatory Factors (GRF), with an index number. The total number of 14-3-3 isoforms is different in various plant species. There are 13 isoforms in
Arabidopsis thaliana [15], 17 isoforms in tobacco
Nicotiana tabacum[16], 25 in banana
Musa acuminate[17] and 7 in a model grass
Brachypodium distachyon[18]. Hierarchic classification of subfamilies is summarized in the
Figure 1
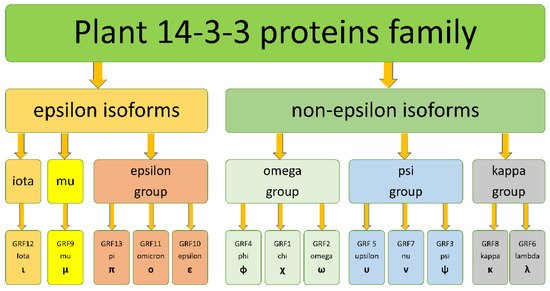
Figure 1. Arabidopsis-based hierarchic classification of plant 14-3-3 proteins subfamilies. All plant 14-3-3s subdivided into two types of isoforms: epsilon and non-epsilon. At the next level of hierarchy there are six subfamilies: iota, mu, epsilon, omega, psi and kappa. In Arabidopsis, most of subfamilies include several isoforms, named by Greek letters or GRFs (General Regulatory Factors).
To study evolution of plant 14-3-3 proteins, we sampled 46 plant species from different branches of the angiosperm phylogenetic tree. The total number of 511 plant sequences broke into two major clades with high (0.99–1) support (Figure 2), 163 in an epsilon clade and 292 in a non-epsilon clade. There were three subclades in the epsilon clade: mu (74), iota (51) and epsilon groups (55), including epsilon itself, omicron and pi isoforms. The mu subfamily was a sister to the epsilon subfamily, whereas iota had a basal position in the epsilon clade. Non-epsilon isoforms included three groups: omega (114), psi (117) and kappa (77). The omega group included phi, chi and omega; the psi group included upsilon, nu and psi; the kappa group included kappa and lambda isoforms.
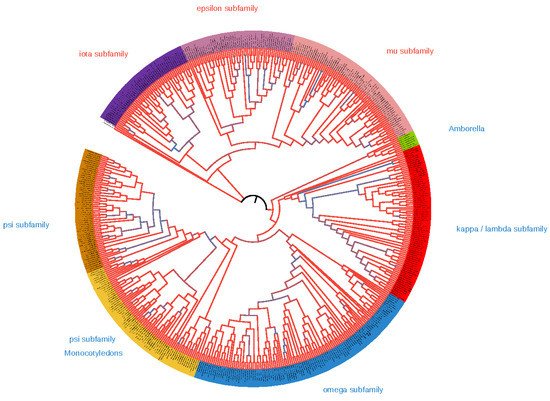
Figure 2. Phylogenetic tree (cladogram) of plant 14-3-3 proteins family based on Bayesian analysis of coding nucleotide sequences. Branches are coloured according to Bayesian support values, maximal support is red, minimal support is blue. Section colours indicate subfamilies. Text colours indicate epsilon (red) and non-epsilon (blue) types of isoforms. Tips of branches are omitted. Full phylogenetic tree in high resolution with all analysed 14-3-3 proteins names is presented in
Supplementary materials for doi.org/10.3390/plants10122724 (Figure S1).
Subfamilies of 14-3-3 proteins were distributed between plant species in an uneven manner. Almost all sampled Eudicots as well as Amborella had isoforms from the iota subfamily, whereas amongst monocots only banana had one iota 14-3-3 protein. There were no iota isoforms in pineapple. Grasses had only the epsilon isoform itself and had neither iota, nor mu isoforms. Some dicot species had several paralogous isoforms from iota and mu subfamilies; among these are apple, soybean, poplar, willow, flax, and aquilegia. Epsilon isoforms were absent in species from the Fabaceae family: beans and medic. Isoforms pi, omicron and epsilon are paralogs belonging to A. thaliana. It was possible to determine orthologous genes for each of three these isoforms only in Brassicaceae, whereas in other taxonomic groups we should imply a joined epsilon–omicron–pi subfamily, or epsilon group. The same diversification pattern was observed for non-epsilon types of isoforms: omega group, psi group and kappa group. The omega group was the most widespread subfamily of plant 14-3-3 proteins. We did not find omega-group isoforms only in banana and Amborella. The majority of species had several duplicated isoforms from the omega subfamily. The psi group was the biggest subfamily of 14-3-3s, particularly many isoforms from the psi group were found in Poales (56 isoforms in 12 species). Seven isoforms were found in wheat and maize, five in each foxtail species. Kappa/lambda isoforms were present in all species except grasses.
Based on phylogenetic relationship, we allocated 14-3-3 isoforms among the main subfamilies (see in
Supplementary materials for doi.org/10.3390/plants10122724: Table S3). Separations of phi, chi and omega in the omega group, upsilon, nu and psi in psi group, kappa and lambda in kappa group, took place only in the Brassicaceae lineage. Thus, we can use these Greek names for other
Arabidopsis species,
A. lyrate and
A. halleri, as well as for other Brassicaceae, but not for other plant lineages. For instance, plants from the Solanaceae family (tomato, potato, tobacco) have at least four isoforms in the omega group. Diversification of these four isoforms in the Solanaceae lineage and diversification of three (omega, phi and chi) isoforms in
Arabidopsis were independent events.
3. The origin of 14-3-3s diversity in Angiosperms
The diversity of isoforms of 14-3-3 proteins in plants is a result of gene duplication events. There were at least 106 whole-genome duplications (WGDs) in angiosperm evolution[19], and a lot of small-scale genome duplications (SSDs) in various plant lineages[20]. The early duplication event occurred in a common ancestor of green plants and lead to formation of pro-epsilon and pro-non-epsilon isoforms. Pro-epsilon probably was close to modern iota isoforms, whereas pro-non-epsilon is more similar with modern kappa isoforms. More late duplication events lead to the formation of iota and epsilon isoforms from pro-epsilon, and kappa and psi from pro-non-epsilon.
The origin of epsilon, pi, omicron, upsilon, nu, psi, chi, phi, omega, kappa and lambda 14-3-3 proteins in Arabidopsis, explained by two recent WGDs, took place in common ancestor of Brassicaceae family circa 14 and 80 million years ago [32,34,35]. At least three duplications produced high diversity of psi-group isoforms in Poaceae[21]. The vast variety of 14-3-3s in banana (25 isoforms)[17] could be driven by a combination of the WGD that happened 110–135 million years ago and three independent lineage-specific WGDs which happened 65–100 million years ago after the separation of banana’s ancestor from Poales[22].
Most of the 14-3-3 family gene duplications could be explained by WGD events, which happened many times during repeated cycles of “hybridization-polyploidization-secondary diploidization”, typical for plant evolution[23]. However, the variety of plant 14-3-3 proteins observed in angiosperms plants is explained not only by duplication, but also by lineage-specific gene loss. For example, the Poaceae family (grasses) lost mu and kappa isoforms, and all Poales (grasses and pineapple) lost the iota subfamily, and the Fabaceae family lost the epsilon-group isoforms. During the cycles of “hybridization-polyploidization-secondary diploidization”, polyploidization is often followed by loss of genetic materials, from individual genes to whole chromosomes.
4. Functionla groups of 14-3-3s vs. their evolutionary origin
14-3-3 proteins play a fundamental role in the regulation of plant physiology, thus there is a question of how species cope with a loss of whole 14-3-3 subfamilies. It is still unknown if 14-3-3 proteins are involved in physiological function in an isoform-specific manner. In an interactome study on developing A. thaliana’s seeds, 27 non-specific proteins were found to interact with 14-3-3s, 28 chi-specific (non-epsilon group) and 49 epsilon-specific[24]. Non-epsilon isoforms omega, kappa, lambda and chi better bind with plasma membrane H+-ATPase than epsilon, mu and omicron isoforms[25]. Different isoforms of 14-3-3 proteins show variation in binding activity with the C-terminus of plasma membrane H+-ATPase. Evolutionarily close phi and omega isoforms demonstrate an opposite capability for binding H+-ATPase[14]. The epsilon isoform in A. thaliana works as a positive regulator in cold-stress adaptation, lambda works as negative[26][27], but the epsilon-like isoform in rice is not affected by low-temperature stress [28].
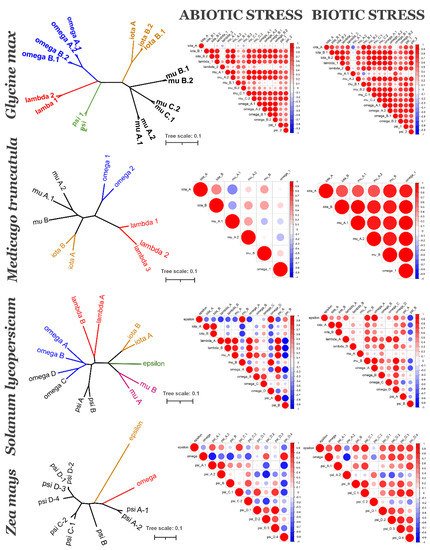
Figure 3. 14-3-3 isoforms of four species: Glycine max (soybean), Medicago truncatula (barrel medic), Solanum lycopersicum (tomato) and Zea mays (maize): phylogenetic relationships (left graph) and coexpression under abiotic and biotic stress (heatmaps). Left graph is unrooted NJ tree of 14-3-3s, bar is number of nucleotide changes per site. Colour of a branch reflects different subfamilies groups. Correlation heatmaps show PCCs between each pair of 14-3-3s. Positive correlations are displayed in red, negative correlations are displayed in blue. The size of the circle and the colour intensity are proportional to the PCCs.
To understand if evolutionarily related 14-3-3 proteins are also functionally related, we analysed their coexpression using PLANEX and EXPath 2.0 databases. Coexpression data shows that each species has its own coexpression pattern for 14-3-3 proteins. The more diverse taxonomically they are, the more different functional groups formed 14-3-3 proteins (Figure 3). In A. thaliana (Figure 4), closely related isoforms from omega, psi and kappa groups had similar coexpression patterns under almost all physiological conditions. On the other hand, pi and omicron, which are closely related to epsilon and included in the epsilon group, showed negative correlation in expression with epsilon. In other species, closely related isoforms were often coexpressed, but not always (Figure 3). The suggestion is that lineage-specific gene loss (such as loss of epsilon in Fabaceae and loss of mu and iota in Poaceae) could be compensated by defunctionalisation of remaining isoforms.
Figure 4. Arabidopsis thaliana 14-3-3 isoforms: phylogenetic relationships (upper graph) and coexpression under different physiological conditions (correlation heatmaps). Upper graph is unrooted NJ tree of 14-3-3s from A. thaliana, bar is number of nucleotide changes per site. Colour of a branch reflects different subfamilies groups. Correlation heatmaps show PCCs between each pair of 14-3-3s. Positive correlations are displayed in red, negative correlations are displayed in blue. The size of the circle and the colour intensity are proportional to the PCCs.
This entry is adapted from the peer-reviewed paper 10.3390/plants10122724