1. Introduction
Cardiovascular disease, predominantly MI, is attributed the highest mortality rate worldwide [1]. Reduced contractility and function, irregular left ventricle remodeling, and uneven stress distribution in the heart muscle are among the complications occurring post-MI, eventually resulting in catastrophic heart failure. According to the American Heart Association (AHA)’s “Heart Disease and Stroke Statistics—2021”, the prevalence of CVD (including heart failure, hypertension, and stroke) in the US population is 49.2% in the age range of 20 years and above [2]. In 2014, 150,000 people died due to MI; thus an estimated approximately 14% of global death occurs mainly due to MI. Furthermore, MI survivors are also 15 times more likely to develop post-disease complications that lead to heart failure, and are prone to die sooner rather than later compared to the normal population [1]. Cardiac ischemia-related deaths have also ascended to the top of the list of causes of death in India, the United States, and Europe, apart from MI [1][3][4]. After MI incidence, male and female patients above 45 years of age have a lower life expectancy, of 8.2 and 5.5 years, respectively [1]. Socioeconomic burdens such as health care infrastructure and treatment costs (USD 11.5 billion) have made MI one of the top ten most expensive illnesses in the United States [1][5]. Researchers and clinicians around the world have been working extensively to reduce the global incidence of MI and develop significant cost-effective treatment strategies to reduce the mortality rate from MI.
The human heart is a complex organ composed of various types of cells such as cardiomyocytes (CM), fibroblasts, endothelial cells, valve interstitial cells, and resident cardiac stem cells. The cells of the heart are very active metabolically, as it physiologically requires adenosine tri-phosphate (ATP) for its function. Nonetheless, the heart lacks endogenous repair or regeneration potential, thus it remains devoid of regenerative capacity. Any defect in size or deficiency in cardiomyocyte numbers leads to life-threatening MI-related cardiovascular complications [6]. Currently, mitigation of CVDs by pharmaceutical drugs and other clinical practices have effectively improved the patient’s survival and quality of life after tissue damage [7]. However, this remains only a short-term solution of temporary duration; the permanent curative would be via heart transplant. Severe shortage of donor organs, post-graft complications, and the limited efficacy of pharmacological interventions has placed the emphasis on cell-loaded scaffold-based therapeutic approaches for cardiovascular complications (CVDs).
The emergence of cardiac tissue engineering (CTE) has not only given substantial hope for resolving or rescuing the damaged heart after MI but also for prompting the regeneration of the damaged myocardium, thus providing a permanent curative. The idea of CTE was first impelled in 1995 by in vitro-generated cardiac tissue obtained from embryonic chicken CMs. This further ushered in the prospect of new research areas around CTE, mainly idealised to translate the bench to bedside. CTE primarily aims to recapitulate the in vivo cardiac niche under in vitro conditions. Therefore, the long-term goals of CTE are considered the construction of in vitro-fabricated tissues for in vivo cardiac repair and regeneration, in vitro preclinical models for evaluation of drug toxicity, and disease models for understanding the development and pathophysiology of heart-related disorders [8]. With the global rise in CVD cases, it is essential to reinforce the treatment modalities for better disease management. In the current scenario, the previously mentioned CTE is considered as at the forefront; however, the conducting of numerous clinical trials is crucial in prioritizing CTE in clinical practice.
Delivery of an engineered scaffold loaded with stem cells, CM mitogens, or pharmacological molecules directly to the infarcted site via either the intra-coronary or intra-myocardial mode leads to the relatively prompt recovery of the infarcted tissue, followed by regeneration and regaining of functional significance. Still to be addressed are the current roadblocks to successive clinical utilization, such as an optimized protocol for stem cell-derived CMs and their source, biomaterials for CM cultures, as well as their delivery strategies [9]. Advanced delivery approaches using injectable or patch-based methods are recently gaining significant attention due to their complexity in design and versatility in application. The most advanced technology, using iPSC-derived CM-loaded microfluidic devices, has now been providing unprecedented opportunities to understand the mechanisms of MI development. This technology can also be employed to study the effects of drugs in the preclinical drug screening phase [10].
The recent technological advances in cardiac tissue engineering, for example, cell-based therapy and patch-based therapy, have been elucidated in Figure 1.
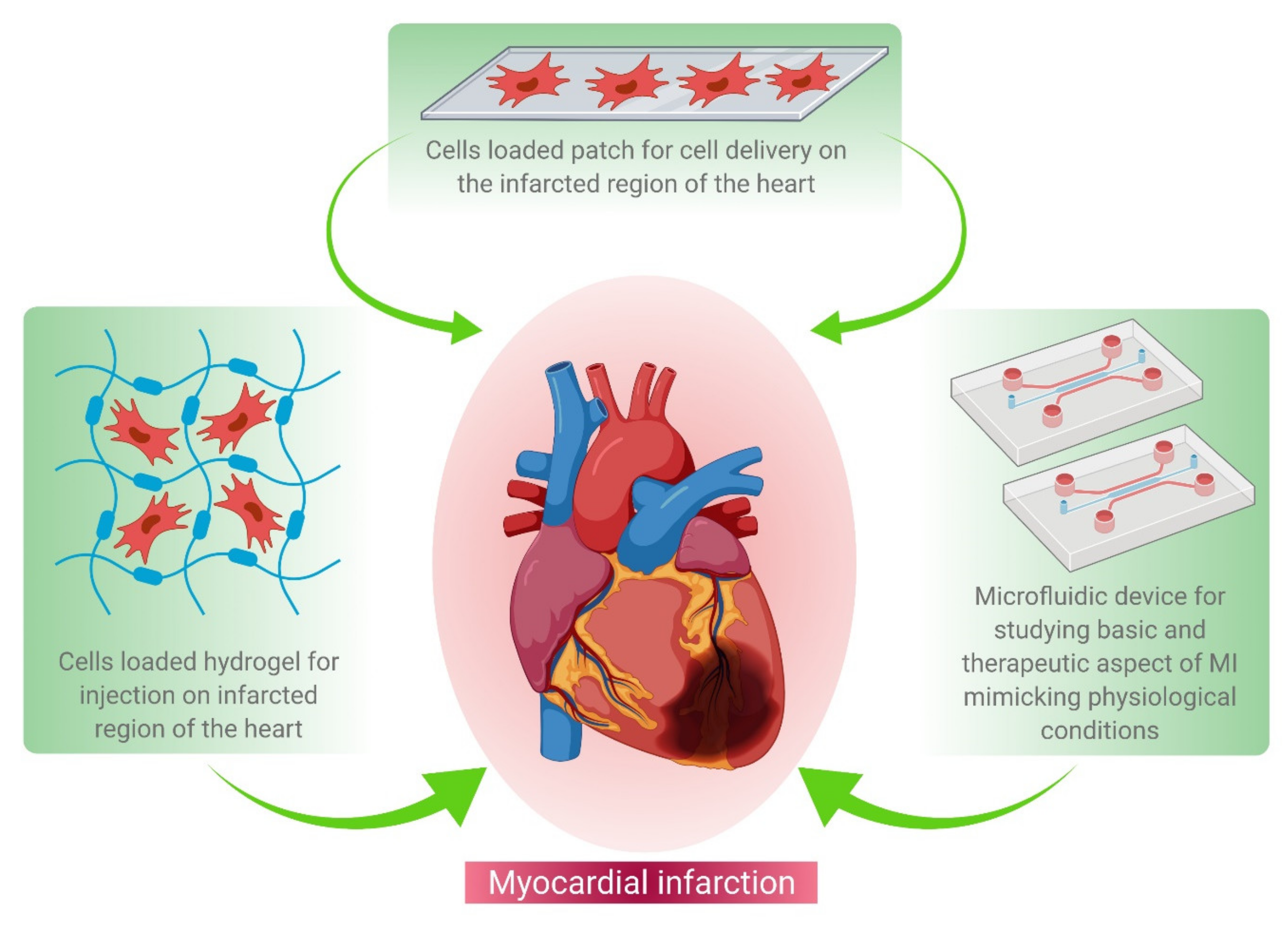
Figure 1. Schematic representation of various tissue engineering approaches for MI treatment. These approaches include hydrogel-based cell delivery (left hand corner), patch-based cell delivery (middle panel), and microfluidics-based drug screening (right corner) during the regenerative therapy of damaged heart tissue.
2. Recent Advances in Cardiac Tissue Engineering for the Management of Myocardium Infarction
2.1. Regenerative Therapy
Heart failure due to the progressive complications of MI mainly occurs due to the limited intrinsic regenerative potential of the myocardium. A left-ventricular assist device (LVAD), fixed internally to relieve the pressure on the heart left region, is the only available medication for the management of post-MI complications. This temporarily delays post MI complications; meanwhile, in such cases, a heart transplant can be the only permanent solution. However, the lack of organ donors has led researchers and clinicians to contemplate alternative therapies available immediately after MI for minimizing cardiomyocyte damage and thereby preventing subsequent heart failure. Under these conditions, cell-based therapy is ideal for repairing the initial injury, restoring lost cardiomyocytes, and preventing the development of a scar (which impairs cardiac function) (
Figure 2). Many research groups are currently investigating the possibility of restoring cardiac function by replacing lost cardiomyocytes or via rejuvenating the resident cardiac stem cell population to counterbalance the lost CMs. Cell therapy has conventionally been a modest procedure in which cells are directly injected into the myocardium
[11][12][13][14][15][16][17][18][19][20]. These cells may function in various ways, including differentiation into cardiomyocytes, support for endogenous regeneration, and/or protection of the affected cells. Several cell types have been investigated for their regenerative ability, each with its own set of benefits and side effects
[13]; among these are mesenchymal stem cells, bone marrow cells, and cardiac progenitor cells. These have been shown to improve heart function in preclinical studies and are currently being studied in clinical trials
[21]. Cell therapy using mesenchymal stem cells and bone marrow cells has been effective to some extent, but these cells are unable to differentiate into cardiomyocytes due to their restricted differentiation potential.
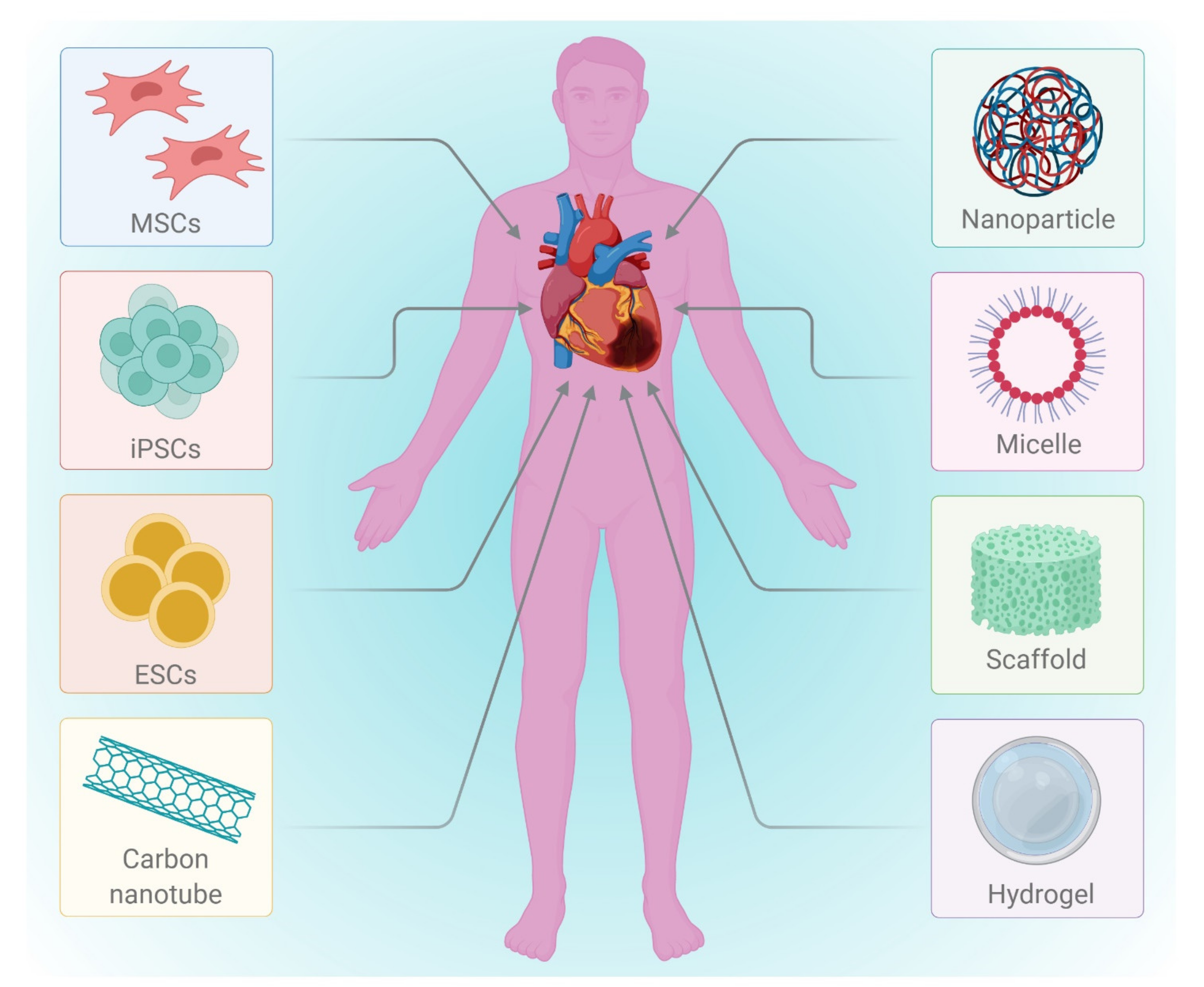
Figure 2. Illustration of stem cell-based regenerative medicine in MI treatment. Various stem cells like MSCs, ESCs, and iPSCs have been employed in MI treatment. Stem cells are delivered through engineered novel biomaterials (via encapsulation) that mimic the native niche. The stem cell-based regenerative therapy is beneficial to either replace the injured area or the whole organ.
2.2. Cell Based Therapy
In the tissue engineering and regeneration process, various types of cells are involved (
Figure 3). Before using any cells, the key issues such as administration of immunosuppression and disease transmission to the host have to be addressed. Although autologous cell transplantation circumvents the use of immunosuppressants and holds a lower risk of disease transmission, the restricted supply hinders its application. Allogenic cells can also be used, but they require immunosuppression and pose a danger of disease transmission. Other disadvantages include the difficulty of collecting cells from donor sources, and of expanding their prior integration into the host. Furthermore, depending on the source of extraction (e.g., elderly persons or diabetic patients), autologous cells may have limited proliferation and differentiation
[13][22][23]. Pluripotent stem cells such as embryonic stem cells (ESCs) and induced pluripotent stem cells (iPSCs) are cells that have the ability to self-renew and to give rise to any of the three primary germ cell layers, but not extra-embryonic tissues
[24]. Studies employing stem cells in an animal model of cardiac injury and their outcomes have been summarized in
Table 1.
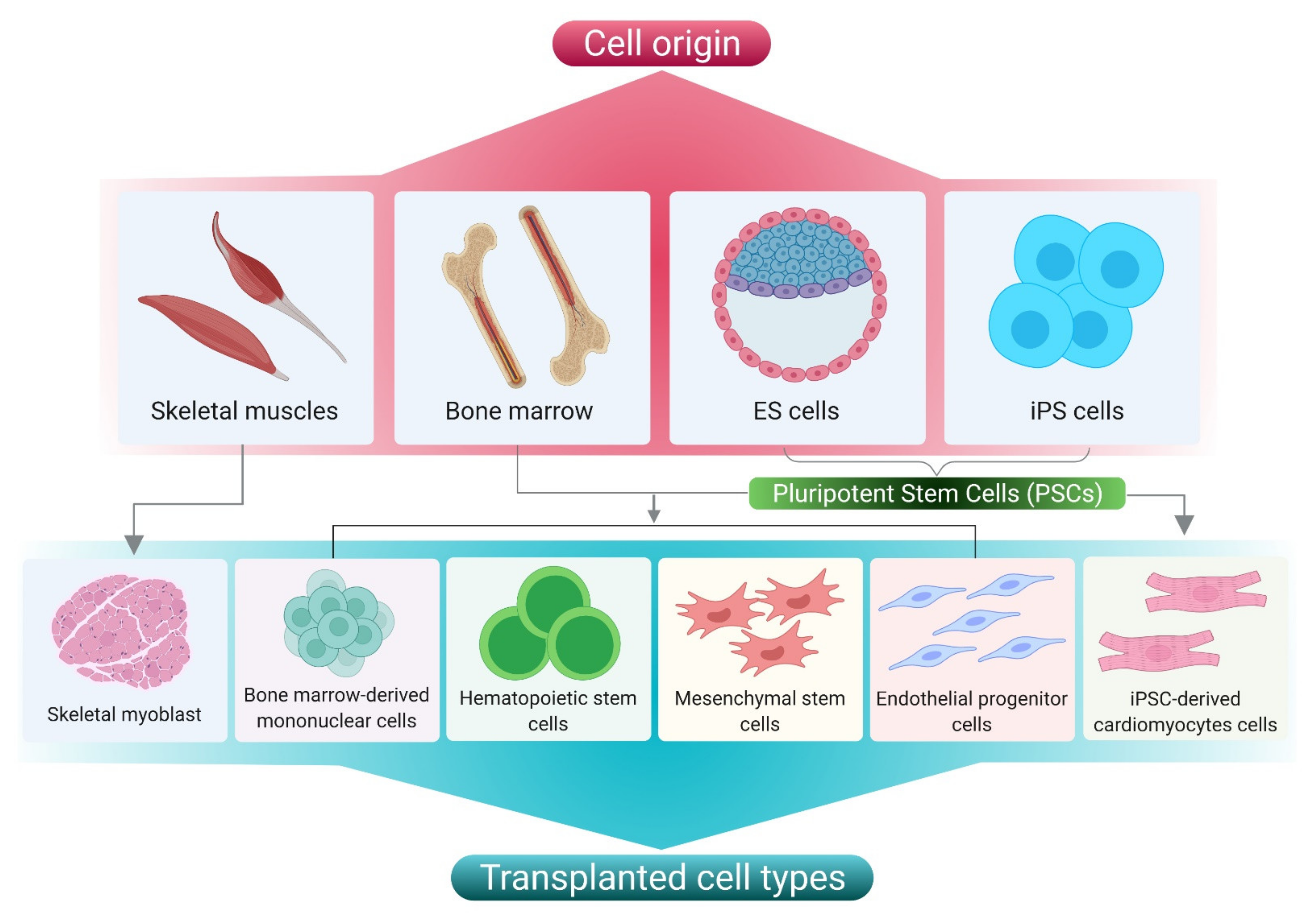
Figure 3. Distinct cells of different origins which are used in the regenerative medicine depicted herein. Depending on the pluripotency, the transplanted cell types can differentiate into various other cells such as skeletal myoblasts, endothelial progenitor cells, chondrocytes, adipocytes, or cardiomyocytes.
Table 1. Description of the cells delivered to the heart by injection. This cell delivery approach has used various cell types including ESCs, iPSCs, MSCs, and CSCs.
Initial Cell Type |
Target Cell Type |
Composition of Delivery Vehicle |
Mode of Delivery |
Animal Models |
Outcomes |
Limitations |
References |
iPSCs |
CMs |
Polyethylene glycol hydrogel |
Trans-epicardial |
MI in nude rats |
Increased infarct thickness and improved muscle content |
No donor cell engraftment was observed |
[25] |
Mouse ESCs |
CMs |
PA-RGDS based gel |
Trans-epicardial |
Mice |
Engraftment and integration of mESC-CMs into host myocardium improved cardiac function |
No information available on cardiac remodelling |
[12] |
iPSCs |
CMs |
PBS solution |
Trans-epicardial |
Post-infarcted swine |
Enhanced angiogenesis, reduced apoptosis, and blunted cardiac remodelling |
No detailed information available on the engraftment of donor cell |
[26] |
MSCs |
**** |
Self-assembling peptide hydrogels (3-D Matrix, Ltd.) |
Surface immobilization by spreading |
Lewis rats |
Augmented microvascular formation and reduced interstitial fibrosis |
No detailed information available on the engraftment of donor cell and CMs differentiation from MSC |
[27] |
MSCs |
**** |
Si-HPMC |
Trans-epicardial |
Lewis rats |
Short-term recovery of ventricular function and attenuated mid-term remodelling |
No detailed information available on the engraftment of donor cell and CMs differentiation from MSC |
[28] |
c-Kit overexpressing CSCs |
**** |
PBS solution |
Intracoronary |
Fischer 344 rats |
Preserved LV function and structure |
Increased cell dose was found to be harmful. Cell tracing or engraftment were not available in detail |
[28] |
CSCs |
**** |
Matrigel and dimethylpolysiloxane mixture gel |
Trans-epicardial |
NOD-SCID mice |
Improved long-term retention of CSCs, cardiac structure and function |
Cell tracing or engraftment were not available |
[29] |
2.3. Microfluidics Based MI Research
Microfluidics deals with the handling of very small volumes of liquid, typically in a length scale of a few micrometers. Since its development in the early 1980s, it has been discovered to be an effective tool with a wide range of applications, from inkjet printers to LOC systems. Over the last two decades, microfluidics has been used in many biological fields such as genomics and proteomics, point-of-care diagnostic systems, biohazard detection, and more. Its recent developments have enabled tissue engineering research to be done in a more cost-effective and highly sensitive way. Research related to MI has been explored using microfluidic devices as it becomes feasible to create a 3D tissue structure and study it in a dynamic condition mimicking both healthy and pathophysiological states of the heart
[30].
Microfluidics emerged from the conventional silicon and glass micro-machining process. With the advent of photo-lithography and other BioMEMS fabrication techniques, the whole process has become more user-friendly. The range of materials used for fabrication is vast, and includes different polymers, silicon, silicon-based materials, metals, etc. Materials are selected based on properties like rigidity or flexibility, optical transparency, biocompatibility, and reactivity to reagents. PDMS is a common material used for microfluidics because of properties like high oxygen permeability, ease of fabrication, and biocompatibility. As cell behavior also depends upon the topography of its environment, the surface chemistry of these materials plays a vital role in cell culture. The biocompatibility of these surfaces can also be increased via processes such as plasma deposition
[31].
Cell culture work close to the physiologic environment is possible in a microfluidic chip, with better control over the process parameters. Such work has led to the use of human samples for research and reduced dependence on animal models for drug discovery and therapeutics. The small sample size requirement for microfluidic devices helps in handling costly samples and reagents. Miniaturized 3D tissue models can produce better studies on physiological systems and their behavior for toxicity assay studies. The flow conditions in a microfluidic device are very much essential in cellular studies, as the physiological environment is seldom static.
The microfluidic LOC device has also been designed to easily generate different physiological, mechanical, or electrical forces on the culture, which are complicated in macro bioreactors. The whole system can be designed and optimized through different available computational software (e.g., Comsol, Ansys, etc.). These devices can also be linked to external analytical devices in order to study real-time cell behavior (
Figure 4)
[32].
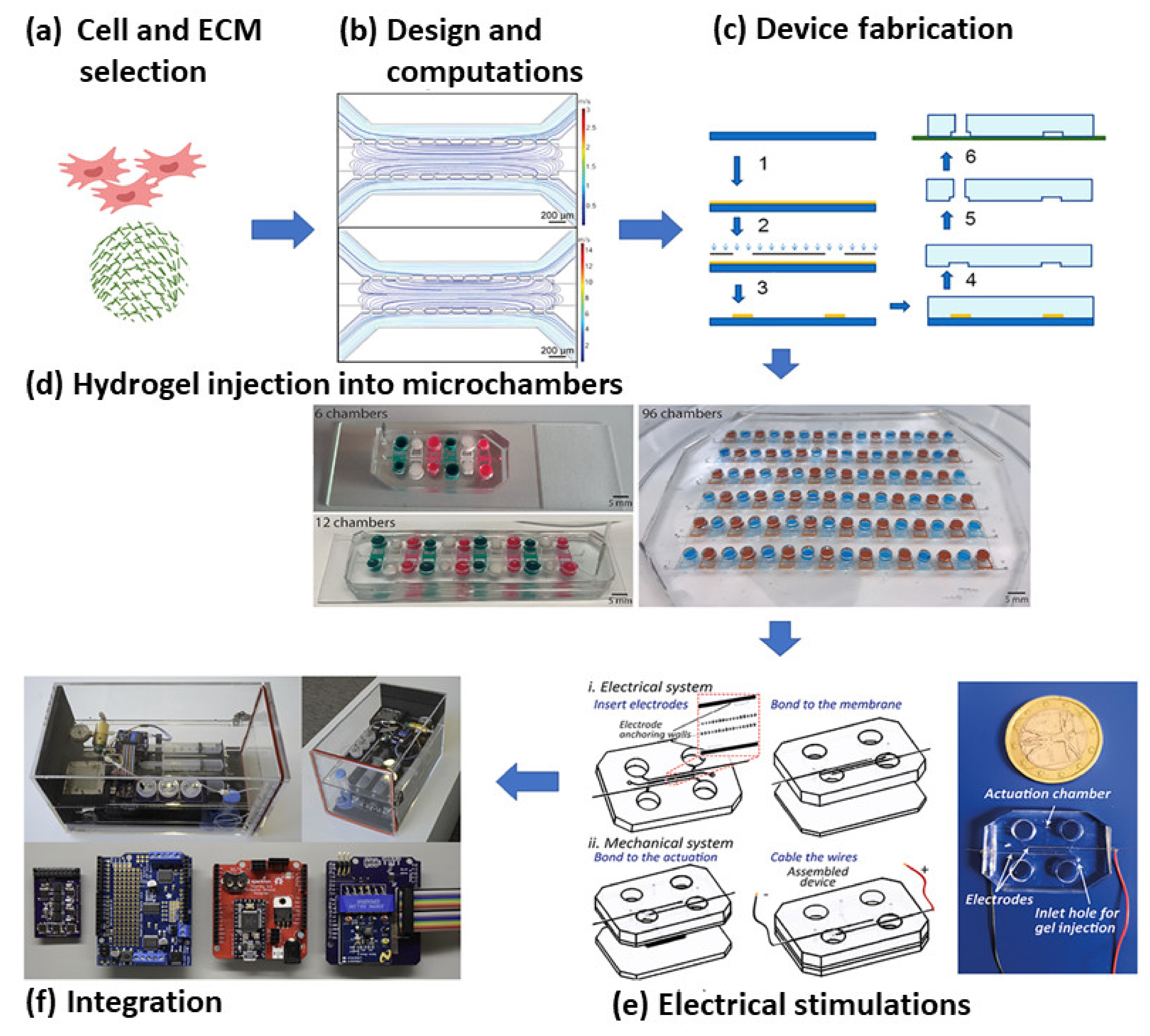
Figure 4. Steps in microfluidic cardiac model generation—(
a) Selection of cells and extracellular matrix are performed based on the physiology to be studied; (
b) this is followed by design of the chip through different computational software to achieve the desired flow contours; (
c) based on the design, the device is fabricated by various microfabrication procedures (the most common being photolithography; the steps of which are 1. spin coating of clean silicon wafer, 2. UV exposure with a mask, 3. dissolution of unwanted resist with developer solution to generate the master pattern, 4. PDMS mold creation from the master pattern, 5. punching of required inlet and outlet holes in the PDMS mold, and 6. bonding of the PDMS mold with a glass plate or another PDMS slab to close the device); (
d) introduction of cell-laden hydrogel into the device for 3D culture (its selection is based on the mechanical properties needed for the micro tissue under study; (
e) completion of the electrical circuit required for stimulation of the cardiac cells; (
f) integration of the device with external circuitry and pumping mechanism for seamless operation of the chip
[33][34][35][36].
3. Future Directions
In spite of technological advances in the study of cell biology, cellular behavior, and the pathophysiology of MI, there are many of unconnected questions that have to be solved in order to achieve a more efficient and effective stem cell-based therapy for MI. Understandably, no single therapeutic approach will suit all, but to do what can be done to narrow down stem cells, delivery approaches, and biomaterials to the minimal and most efficient formation is the major challenge requiring a detailed and thorough exploration. However, it is still not clear which stem cell is ideal for treating acute or chronic MI. Which mode of delivery is best or better than others, and which biomaterial is most suitable for stem cell delivery to the infarcted region? Many clinical trials have been completed so far, and many will be conducted in the upcoming years. However, a clear strategy has to be evolved in order to find the best combination of stem cells, delivery approach, and biomaterial components to meet the requirement in most cases, if not all.
Microfluidics is another major research area that has helped in the resolution of many complicated questions such as the selection of biomaterials and the mechanisms involved in transplanted stem cell-mediated MI heart regeneration, as well as the basic pathophysiology of MI. Most importantly, microfluidics can be used to more efficiently understand the angiogenesis process and the therapeutic interventions required to increase the vasculature density in the infarcted region. Eventually, it could help to improve the functionality of the infarcted heart.
Stem cell delivery to the infarcted myocardium has become indispensable for regenerating the heart so as to regain functionality similar to native cardiac function. To this end, various stem cells have been used for delivery to the infarcted heart. Processes used include direct injection into the myocardium and using a 3D biocompatible scaffold (commonly called a patch) to deliver a higher dose of cells with uniform distribution across the infarcted region. Several mechanisms have been documented where either the transplanted stem cells differentiate into functional CMs and integrate with the heart to improve cardiac function or act through a paracrine manner to induce the regeneration of CMs, increase neovascularization, reduce scar formation, increase ventricular function and decrease remodeling of the myocardium. Hence, the forthcoming mechanistic studies on exosomes and direct tagging of stem cells for monitoring the homing studies after transplantation may further result in translating bench studies to the bedside.
This entry is adapted from the peer-reviewed paper 10.3390/cells10102538