2. Methane Flux from Mangrove Forests
2.1. Significance of Methane Emission from Mangrove Forests
2.2. Factors Associated with Methane Emission
2.2.1. Soil Conditions
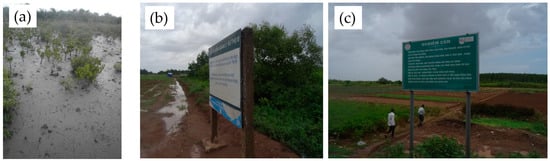
Mangrove forests sequester a significant amount of organic matter in their sediment and are recognized as an important carbon storage source (i.e., blue carbon, including in seagrass ecosystems and other coastal wetlands). The methane-producing archaea in anaerobic sediments releases methane, a greenhouse gas species. The contribution to total greenhouse gas emissions from mangrove ecosystems remains controversial. However, the intensity CH4 emissions from anaerobic mangrove sediment is known to be sensitive to environmental changes, and the sediment is exposed to oxygen by methanotrophic (CH4-oxidizing) bacteria as well as to anthropogenic impacts and climate change in mangrove forests. This review discusses the major factors decreasing the effect of mangroves on CH4 emissions from sediment, the significance of ecosystem protection regarding forest biomass and the hydrosphere/soil environment, and how to evaluate emission status geospatially. An innovative “digital-twin” system overcoming the difficulty of field observation is required for suggesting sustainable mitigation in mangrove ecosystems, such as a locally/regionally/globally heterogenous environment with various random factors.
This entry is adapted from the peer-reviewed paper 10.3390/f12091204