1. Introduction
Within ILs, there are electrostatic and dispersive interactions at different length scales, leading to a highly anisotropic character. The ions have a large structural diversity which varies from inorganic to organic, simple to complex, including fully or partially ionized acid or base, organic polymeric metal ions, or metalated coordination polymers [1], giving a boundless variety of cation/anion combinations, estimated around the order of 1019 [2].
The first IL (ethylammonium nitrate) was reported in 1914 by Paul Walden, who never expected that ILs would become such an important scientific area a century later. The number of papers on ISI Web published about ILs in the last five years is more than 38,000; this is explained by several factors: ILs are environmentally friendly solvents with properties such as extremely low vapor pressure, low combustibility, excellent thermal stability, and a wide temperature range in their liquid state. The low volatility and combustibility of ILs, along with the possibility of building ionic liquids in which physical and chemical properties can be fine-tuned, has been a reason for their enormous use in recent years
[3][4][5]. They have been extensively used as low-environmental-impact solvents in catalysis and separation techniques, as lubricants and additives, as auxiliaries in analytical techniques, as thermal fluid, and as electrolytes
[6][7][8][9][10][11][12][13][14]. Additionally, a wide variety of ILs are transparent through the visible and NIR (near-infrared) spectral regions, a key property for optical solvents
[15], and today new ILs are designed for specialized applications in optics and soft luminescent materials
[16].
Lanthanides (Ln) are a group of 15 chemical elements, with atomic numbers 57 through 71, all of which have one valence electron in the 5d shell. All of these elements present similar chemical properties, with +3 being the most stable oxidation state, but the +2 and +4 oxidation states are also common
[17] (
Figure 1).
Figure 1. Lanthanide family, excluding Promethium (Pm with atomic number 61 does not exist in nature). Eu (had been covered in a lot of review papers and will not be focused in this entry) is in blue; lighter elements in brown and heavier elements in green.
Most lanthanides present luminescence
[18], and this property has been abundantly explored by studying several lanthanide compounds in different chemical environments. The incorporation of metals into ILs opens the potential to combine additional chemical and physical properties in the ILs. In this light, Ln cations are very interesting for their potential to endow ILs with additional features, as Ln compounds are well known for their outstanding properties, including optical, magnetic, or catalytic activities. Focusing on the luminescent properties of the Ln
3+ cations, they present unique optical features over other luminophores, such as excellent luminescence efficiency, tunable emission (choice of Ln
3+), large pseudo-Stokes shifts, long-lived excited states, and unique monochromaticity
[19]. As such, it is not surprising that Ln compounds have been developed for light amplification and generation, security tags, drug delivery, bioimaging, luminescent sensors, and solar energy conversion, among many other applications
[20]. The luminescence of Ln
3+ ions can arise from intraconfigurational 4f–4f transitions, interconfigurational 4f
n→4f
n5d
1 transitions, and charge–transfer transitions (ligand to metal and metal to ligand). The emission of Ln
3+ ions derived from f–f transition covers the NIR (Pr
3+, Nd
3+, Sm
3++, Eu
3+, Dy
3+, Ho
3+, Er
3+, Tm
3+, Yb
3+) through visible (Pr
3+, Nd
3+, Sm
3+, Eu
3+, Tb
3+, Dy
3+, Ho
3+, Er
3+, Tm
3+) to UV (Gd
3+) regions.
It has long been known that the molar absorptivity of lanthanide ions is very low (ε = 2–12 M
−1cm
−1), but this problem can be easily addressed using intramolecular energy transfer processes commonly known as the “antenna effect”. The “antenna effect” is a concept introduced by S. I. Weissman in the early 1940s
[21], which refers the use of highly absorptive organic ligands coordinated with Ln ions that function as light harvesters. The mechanism consists of intramolecular energy transfer from excited states of the ligands to the Ln ion, which dramatically enhances Ln’s luminescence, even under low excitation energy. A detailed explanation of the sensitization of Ln ions is beyond the scope of this entry, but there are many publications addressing this issue that an interested reader can consult
[22][23].
Figure 2 presents a schematic representation of the antenna effect, as well as its Jablonski diagram including other competing energy transitions within the phosphor molecule.
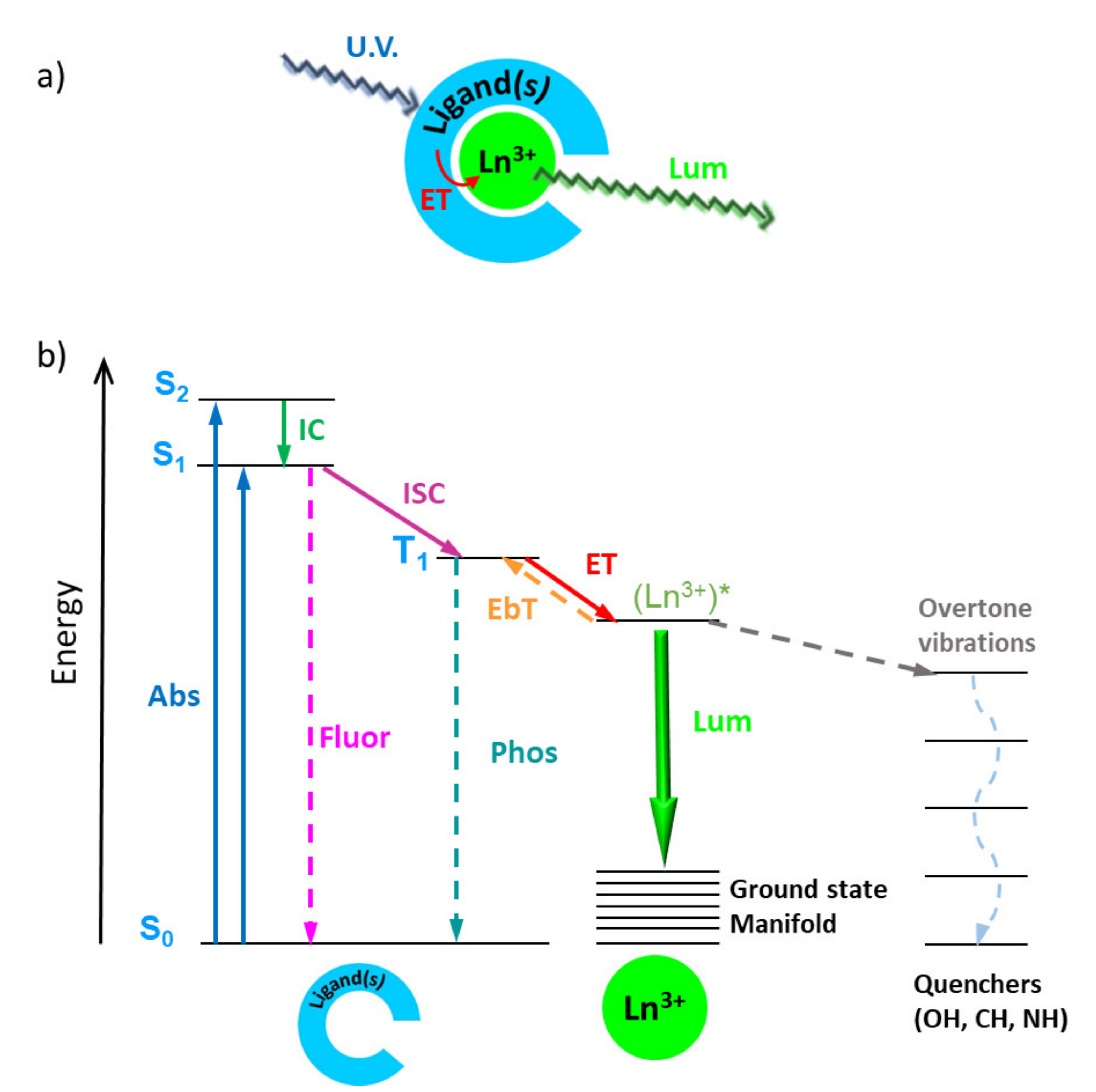
Figure 2. (a) Schematic representation of the antenna effect, and (b) a Jablonski diagram of the antenna effect (solid arrows) together with competing energy transitions (dashed arrows). Abs: absorbance; IC: internal conversion; Fluor: fluorescence; ISC: intersystem crossing; Phos: phosphorescence; ET: energy transfer; EbT: energy back-transfer; Lum: luminescence.
A major problem in Ln emission is luminescence quenching by energy loss through non-radiative relaxation pathways, due to energy transfer to high-energy local-mode vibrations such C–H, N–H or O–H stretching modes in coordinating ligands and solvent molecules. This is particularly relevant for NIR luminescent Ln phosphors due to the narrow energy gap between their lowest excited state and highest ground state levels. Hence, the number of vibration quanta required for their non-radiative deactivation is smaller
[24]. For instance, for Er
3+ compounds, two activated O–H vibrations are enough to enable a radiationless return to the ground state. In the case of Ho
3+-ILs, only one example has been reported to date, by Mudring et al.
[2]. Thus, it is not surprising that NIR luminescence is more sensitive to quenching by water than the visible luminescence of Eu
3+ [25]. For a better visualization,
Figure 3 presents an energy diagram of the NIR luminescent states of Nd
3+, Er
3+, and Yb
3+, with varying numbers of C–H, N–H, and O–H overtones.
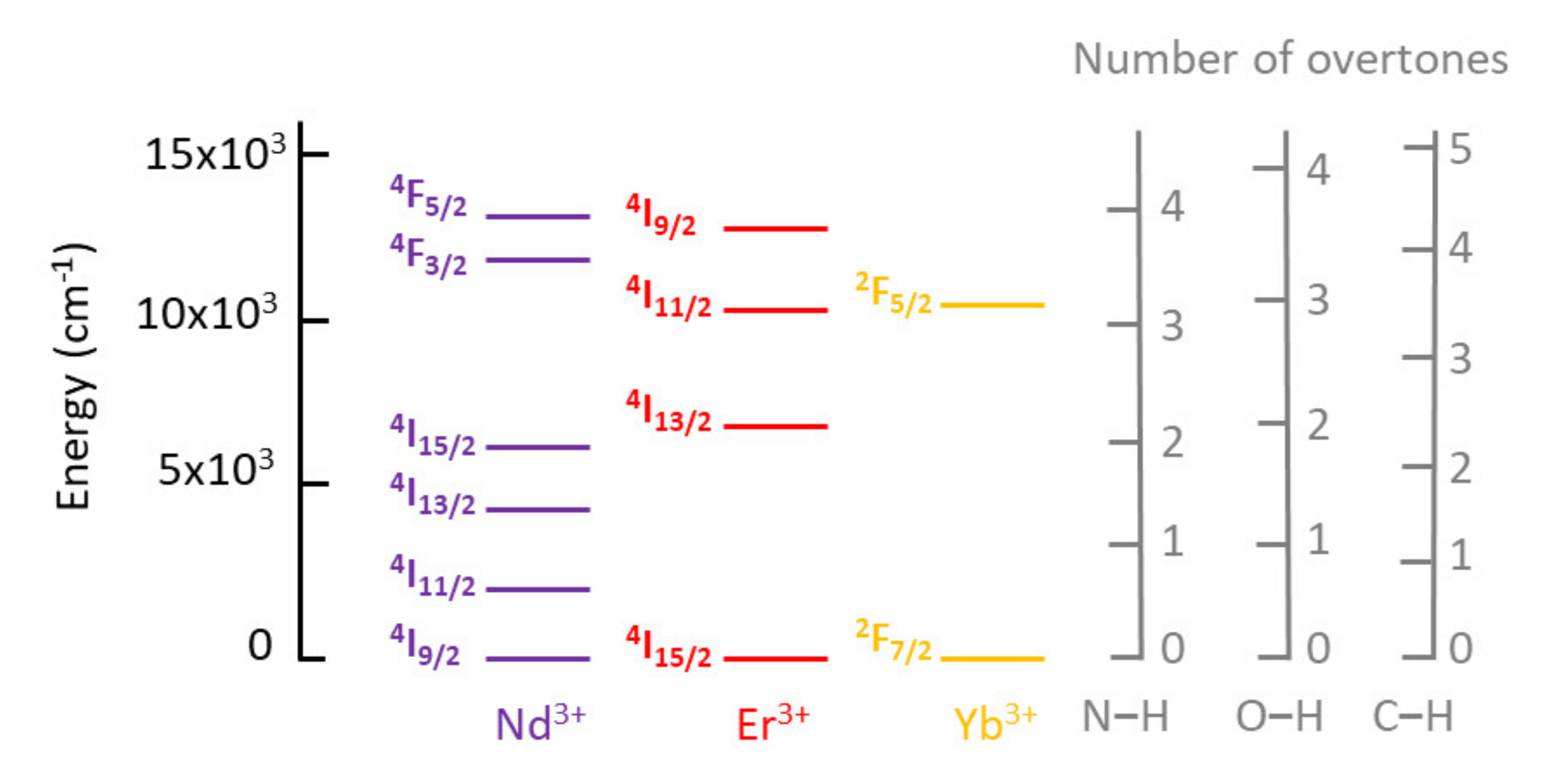
Figure 3. Energy diagram of the NIR luminescent states of Nd3+, Er3+, and Yb3+, and C–H, N–H, and O–H overtones.
In 2010, a review by Eliseeva and Bünzli made a point of the utility of lanthanide luminescence for a variety of functional materials, including “soft” luminescent materials such as liquid crystals, ionic liquids, and ionogels
[26]. A review of lanthanides and ionic liquids, published in 2010 by Mudring and Tang
[27], addressed various topics such as catalysis and luminescence. In a 2013 review, Feng and Zhang approached hybrid compounds by analyzing various properties including the luminescence of compounds containing Ln ions
[28]. Additionally, ionic liquids containing lanthanides (but not exclusively) have been studied for their magnetic properties
[29]. Recently, Prodius and Mudring conducted a review discussing the structural and coordination chemistry of ILs derived from rare earth metals, and their practical applications
[20].
The combination of Ln with ILs has risen as a powerful platform for potential application in several hi-tech processes, screen display technologies, anticounterfeiting technology, heat-storage materials, in situ imaging, optical sensing, energy harvesting, advanced luminescent coatings, etc.
[30][31].
Due to its outstanding emissive properties, europium has received special attention in optical studies based on its luminescence
[32][33][34][35][36][37]. In this entry, the focus will be on the luminescence of ionic liquids/lanthanides other than europium, to which much less attention has been given.
The application of ILs in lanthanide chemistry has raised much interest in recent years. Their initial use was as a simple solvent, but they have been playing an increasing role not only as solvents, but also as reagents, templates, binders, linkers, or property modifiers. The following subsections will address several of these roles in the combination of lanthanides with ionic liquids.
2. Luminescent Ln-Ionic Liquids beyond Europium
To obtain highly efficient Ln molecular light-conversion devices, it is necessary to optimize several parameters: avoid self-quenching channels, use chromophores with high molar absorbance and ideal energy positions of singlet and triplet states (for an efficient energy transfer to Ln3+ ions), while avoiding competitive non-radiative pathways such as multiphonon relaxation to high-energy vibrations (e.g., O–H, C–H, and N–H stretching modes). With this context in mind, the combination of lanthanides and ionic liquids began by using ILs as matrices to protect Ln3+ ions from vibration-induced deactivation processes—mainly from the ever-present water adsorbed in organic solvents. Although ILs proved to provide good protection against the presence of water within the first and/second coordination spheres of the Ln centers, due to the low solubility of the Ln salts, this method usually enabled low concentrations of Ln ions, although higher concentrations could be achieved by the use of the same anionic moieties as both the ligand (of the Ln complex) and the anion (of the ILs). This shortcoming was circumvented by preparing Ln-based ionic liquids, either via direct preparation by metathesis, or by dissolving Ln salts in ILs with anions with coordinating capabilities. Ln-containing ionic liquids proved to be promising materials because, although liquids, they provide a low-phonon environment for the Ln3+ center, leading to appreciable excitation state lifetimes. Typically, liquid-state lanthanide compounds present lower emission quantum yields (Φ) than those in the solid state, due to a less rigid environment and energy loss from collisions. As such, it is not surprising that emission quantum yields for Ln@ILs are very low. The same reasoning is applicable to Ln-ILs although, surprisingly, out of the 58 Ln-ILs presented in our recent review paper (see reference at the end of this entry), only one RTIL—[C6mim]3[Sm(NO3)6]—had its emission quantum yield determined, with a value of 2.73% [38]. Another important aspect to stress is that since Ln@ILs are liquids, no structural characterization was available for the majority of these compounds.
It is worth mentioning that many of the Ln-ILs were studied not only as phosphors, but also as paramagnetic liquids, opening avenues for multifunctional applications. Additionally, the combination of Ln and ILs has aroused so much interest that it led to the emergence of a new field of research, focused specifically on soft materials. In this area, new ionogels have been developed through covalently grafting—or simply dispersing—Ln complexes into silica-based materials, polymer matrices, liquid crystals, etc.
It was not intended to include ionogels in this entry but, since they are becaming more and more importent and just as an example, a simple and environmentally friendly (solvent-free) preparation of ionogels via the incorporation of Ln-ILs within poly(methyl methacrylate) (PMMA) was reported by Wang et al. as early as 2013 [39]. In that work, the ILs Tb(sal)@[Carb-mim][Tf2N] and Eu(tta)@[Carb-mim] [Tf2N] were directly dissolved into MMA monomers with azodiisobutyronitrile (polymerization initiator), with stirring at 80 °C, yielding a yellowish liquid that was then cast into glass slides or glass bottles. After drying, ionogels in the form of monoliths, films, and flexible self-standing films could be obtained ( Figure 14 ).
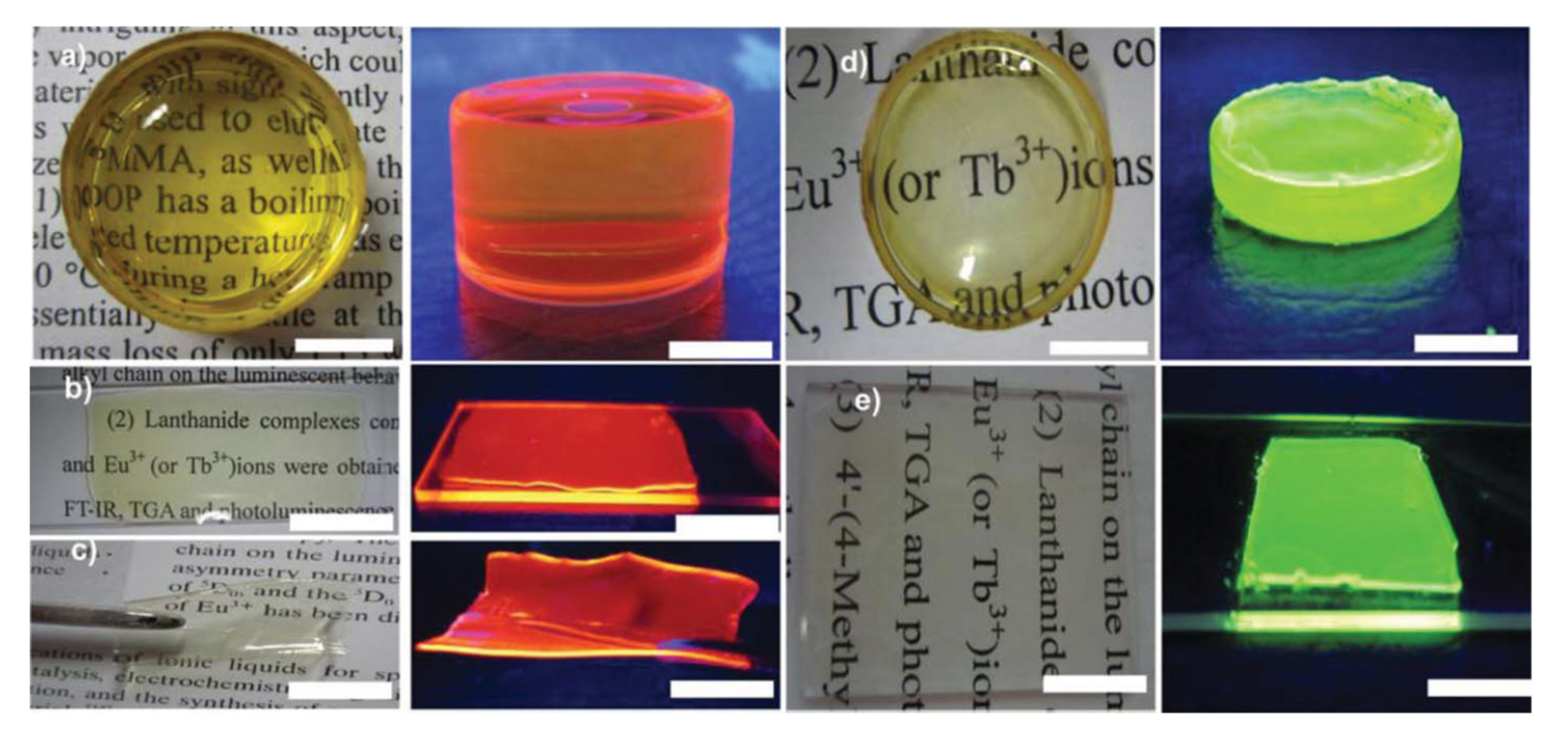
Figure 14. Digital photos of the Ln-ILs-PMMA: (a–c) Eu(tta)–[Carb-mim][Tf2N]@PMMA under daylight (right) and UV light (left); (d–e) Tb(sal)–[Carb-mim][Tf2N]@PMMA under daylight (right) and UV light (left). The scale bar is 1.0 cm. Reproduced with permission from [39].
The accomplishments described in this entry prove Ln-ILs to be outstanding and promising optical materials. However, this field of research is still underdeveloped when compared with other fields of ionic liquid chemistry. Therefore, new studies focusing on different combinations of Ln ions and new ligands will certainly lead to more efficient luminescent molecular devices, paving the way for practical applications as varied as catalysis, biochemical analysis, energy production, and non-invasive diagnostics, such as biolabels.
This entry is adapted from the peer-reviewed paper 10.3390/molecules26164834