1. Introduction
Due to the high energy conversion efficiency and environmental friendliness, hydrogen fuel cells have been regarded as one of the most important clean energy conversion devices to solve the energy crisis and environmental pollution. However, the main challenge in hydrogen fuel cells is to find a highly efficient catalyst for the oxygen reduction reaction (ORR) [1]. The complex multi-step electron transfer process and the slow mass diffusion of the reaction itself lead to slow ORR kinetics [2]. Hitherto, platinum (Pt)-based catalysts are still considered as the most efficient ORR catalysts, but the high cost and poor durability severely limit their wide applications [3]. Therefore, these disadvantages have triggered intense interest in non-Pt catalysts with both high ORR activity and stability. Currently, a variety of non-platinum metal-based ORR catalysts, such as defective carbon materials [4], heteroatom-doped metal-free carbon [5], transition metal/heteroatom-co-doped carbon [6,7], intermetallic compounds [8], and metal nitrides [9], have been synthesized. Among them, two-dimensional (2D) materials with atomic or molecular thickness and infinite planar length exhibit peculiar physicochemical properties, including high specific surface area, adjustable band structure, and electromagnetic properties, which make 2D materials become one of the most important non-Pt-based ORR catalysts. [10–13].
A variety of 2D materials have been found for electrocatalysis. Among heteroatom-doped carbon [14], layered metal hydroxide (LDH) [15], layered metal oxide [16], 2D metal-organic frameworks (2D-MOFs) [17], 2D covalent organic frameworks (2D-COFs) [18], graphite carbonitrides (g-C3N4) [19], hexagonal boron nitride (h-BN) [20], black phosphorous (BP) [21], MXenes [22], and 2D transition metal dichalcogenides (TMDs) [23–25], TMDs are a class of materials with great application prospects and basic research value. The chemical formula of TMDs is MX2, where M represents transition metal elements, including group IV (Ti, Zr, or Hf), group V (V, Nb, or Ta), group VI (Mo, W), group VII (Tc, Re), or group X (Pd, Pt), and X refers to a chalcogen atom (S, Se). In a TMD, X-M-X units stack on each other to form a sandwich structure, and the layers are combined by van der Waals forces [26].
As the thickness decreases, 2D TMDs exhibit a series of special properties. During the conversion process of TMDs from bulk to single-layered 2D materials, significant bandgap transitions occur, resulting in excellent photoelectric performance. Besides, due to the high degree of structural controllability and anisotropy of 2D materials, 2D TMDs can be controlled to form various shapes and sizes, doped with different heteroatoms, and their surfaces can be modified with other materials to obtain a unique crystal structure [27]. More importantly, there are unsaturated coordination and dangling bonds at the edges of TMDs nanosheets. These special properties of TMDs nanosheets provide many inspirations for basic research in many fields including catalysis [28–33], transistor [34], energy storage [35–39] and sensor [40–42]. Among all 2D TMDs, MoS2 is one of the few with a natural layered structure, indicating that MoS2 can be stripped to obtain high-quality 2D MoS2 without complicated chemical synthesis [26]. Therefore, the cost of preparing 2D MoS2 is much lower than other 2D TMDs due to the simple synthesis conditions. More importantly, 2D MoS2 is a 2D semiconductor with a direct band-gap, which has the best electric performance among 2D TMDs [43]. These make 2D MoS2 get more attention among 2D TMDs.
2. Structural Properties of 2D MoS2
The properties of 2D MoS2 are highly dependent on their crystal phase, structure size and chemical composition. In the MoS2 structure, the coordination of S elements and the stacking state between layers can form different electronic characteristics. The most common crystal structures of MoS2 are 1T and 2H phases, where the number represents the number of layers of the S-Mo-S layer in a unit cell, and T and H represent the tetragonal crystal system, and the hexagonal crystal system, respectively [44]. As shown in Figure 1A,in the 2H phase, each Mo atom exists in the center of the hexagonal prism coordination structure and covalently bonded to six S atoms, and exhibits semiconductor properties. In the 1T phase, one Mo atom and six S atoms form a twisted octahedral coordination structure, with metallicity [45]. In MoS2, the S-Mo-S of each planar unit is regarded as a single layer, which is composed of two layers of S atoms and an intermediate layer of Mo atoms [46]. Due to the weak van der Waals force connection between the layers, the peeling between the layers is easy to occur, which makes it possible to synthesize single-layer MoS2 [47].
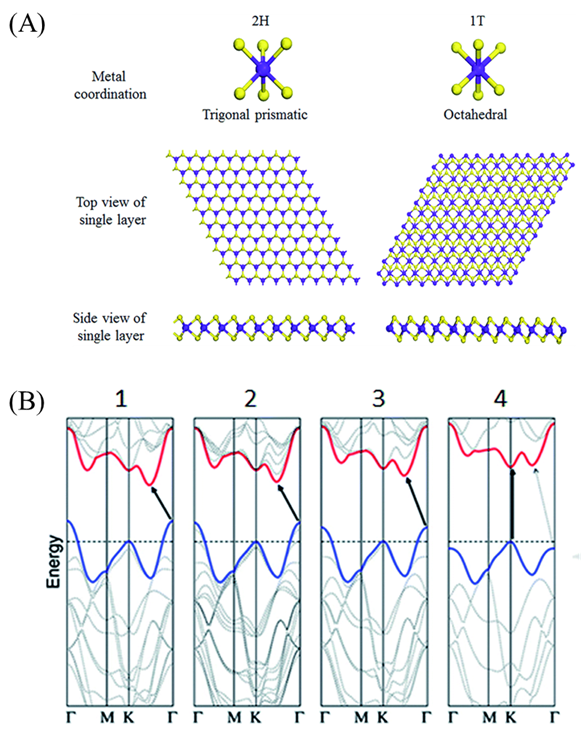
Figure 1. (A) Metal coordination, top view, and side view of single-layer 2H-MoS2 and 1T-MoS2. Reproduced with permission from [44] Copyright © 2021 The Royal Society of Chemistry. (B) Energy dispersion (energy versus wavevector k) in (1) bulk, (2) quadrilayer, (3) bilayer, and (4) monolayer MoS2 from left to right. Reproduced with permission from [48] Copyright © 2021 The American Chemical Society.
As shown in Figure 1B, the bulk material of MoS2 is an indirect-bandgap semiconductor, in which the top of the valence band is located at point G and the bottom of the conduction band is located at the midpoint of the axis of symmetry of G–K, while in single-layer MoS2, the top of the valence band and the bottom of the conduction band coincide with point K, indicating that single-layer MoS2 is a direct-bandgap semiconductor [48]. This indirect-to-direct transition of the bandgap from bulk to the single-layered 2D material is caused by the quantum confinement effect. The electronic properties of 2D MoS2 are closely related to the d electrons existing in the d orbital between the bonding band and the anti-bonding band of the Mo–S bond. In 2H-MoS2, the non-bonded d orbitals are fully occupied, resulting in semiconductor properties. In 1T-MoS2, the non-bonded d orbitals are partially filled, resulting in higher conductivity. As the d-orbital electron occupancy rate decreases, 2D MoS2 gradually changes from a semiconductor to a conductor, which endows the material with different physical and chemical properties [27]. When the thickness of MoS2 drops to the atomic level, a large number of exposed surfaces can undergo oxidation or reduction reactions with various reagents [49]. These surface atoms can escape in the reaction to form vacancy defects, simultaneously leading to the disorder of the nanostructure and a reduction in the coordination number of surface atoms. By adjusting the vacancy defects, we can further modify the electronic characteristics of MoS2. At the same time, unsaturated edges and dangling bonds often appear on the edges of 2D MoS2. These unique edge defects also provide possible active sites for catalytic reactions [2,50].
So far, many 2D MoS2-based materials have been applied to ORRs. At present, it is generally believed that the active site of 2D MoS2 in the ORR is the unsaturated Mo site exposed at the edge. Because the Mo site is positively charged due to the possible polarization effect of the surrounding negatively charged S atoms, it can, therefore, be easily combined with negatively charged O atoms [51]. Simultaneously, the vacancy defects on the basal plane also contribute to ORR performance. In general, an excellent ORR electrocatalyst must have favorable stability, small overpotentials, high current densities, and low manufacturing cost [52]. Although MoS2 has a low cost and high chemical stability, its large inert basal plane and poor electron transport properties limit its catalytic activity. However, it is undeniable that after adjusting the structure of 2D MoS2, it still possesses unique advantages and broad development prospects for ORRs. Here are some advantages of 2D MoS2 in ORRs:
1) The uncoordinated metallic edge centers not only provide a certain intrinsic ORR activity but also give an opportunity for effective functionalization with different groups.
2) Compared with bulk MoS2, nanostructured MoS2 has a smaller particle size and a larger specific surface area. The small size 2D MoS2 is more sensitive to the catalytic reactions compared with bulk MoS2. At the same time, the large surface area can anchor more active sites [53]. Besides, 2D MoS2 has a unique open structure, which makes ORR reactants easily access the active site.
3) 2D MoS2 with a uniformly exposed lattice plane can supply a much simpler condition as an ideal platform for combing experiments and theoretical results, which is helpful to further understand the origin of ORR activity.
References
- Alaswad, ; Omran, A.; Sodre, J.R.; Wilberforce, T.; Pignatelli, G.; Dassisti, M.; Baroutaji, A.; Olabi, A.G. Technical and Commercial Challenges of Proton-Exchange Membrane (PEM) Fuel Cells. Energies 2020, 14, 144, doi:10.3390/en14010144.
- Chen, ; Yang, K.; Jiang, B.; Li, J.; Zeng, M.; Fu, L. Emerging two-dimensional nanomaterials for electrochemical hydrogen evolution. J. Mater. Chem. A 2017, 5, 8187–8208, doi:10.1039/c7ta00816c.
- Yoo, ; Yu, Y.-S.; Ha, H.; Lee, S.; Choi, J.-S.; Oh, S.; Kang, E.; Choi, H.; An, H.; Lee, K.-S.; et al. A tailored oxide interface creates dense Pt single-atom catalysts with high catalytic activity. Energy Environ. Sci. 2020, 13, 1231–1239, doi:10.1039/c9ee03492g.
- Zhu, ; Huang, Y.; Mei, W.; Zhao, C.; Zhang, C.; Zhang, J.; Amiinu, I.S.; Mu, S. Effects of Intrinsic Pentagon Defects on Electrochemical Reactivity of Carbon Nanomaterials. Angew. Chem. Int. Ed. Engl. 2019, 58, 3859–3864, doi:10.1002/anie.201813805.
- Jiang, ; Gu, J.; Zheng, X.; Liu, M.; Qiu, X.; Wang, L.; Li, W.; Chen, Z.; Ji, X.; Li, J. Defect-rich and ultrathin N doped carbon nanosheets as advanced trifunctional metal-free electrocatalysts for the ORR, OER and HER. Energy Environ. Sci. 2019, 12, 322–333, doi:10.1039/c8ee03276a.
- Jin, ; Zhou, H.; He, D.; Wang, Z.; Wu, Q.; Liang, Q.; Liu, S.; Mu, S. MOF-derived 3D Fe-N-S co-doped carbon matrix/nanotube nanocomposites with advanced oxygen reduction activity and stability in both acidic and alkaline media. Appl. Catal. B Environ. 2019, 250, 143–149, doi:10.1016/j.apcatb.2019.03.013.
- Zhang, ; Zhou, T.; Chen, M.; Feng, H.; Yuan, R.; Zhong, C.a.; Yan, W.; Tian, Y.; Wu, X.; Chu, W.; et al. High-purity pyrrole-type FeN4 sites as a superior oxygen reduction electrocatalyst. Energy Environ. Sci. 2020, 13, 111–118, doi:10.1039/c9ee03027a.
- Du, X.; He, Y.; Wang, X.X.; Wang, J.N. Fine-grained and fully ordered intermetallic PtFe catalysts with largely enhanced catalytic activity and durability. Energy Environ. Sci. 2016, 9, 2623–2632, doi:10.1039/c6ee01204c.
- Lou, ; Liu, J.; Liu, M.; Wang, F. Hexagonal Fe2N Coupled with N-Doped Carbon: Crystal-Plane-Dependent Electrocatalytic Activity for Oxygen Reduction. ACS Catal. 2020, 10, 2443–2451, doi:10.1021/acscatal.9b03716.
- Guo, W.; Hu, Z.; Liu, Z.B.; Tian, J.G. Stacking of 2D Materials. Adv. Funct. Mater. 2020, 10.1002/adfm.202007810, doi:10.1002/adfm.202007810.
- Zhang, ; Zhang, J.; Wan, S.; Wang, W.; Fu, L. Stimuli-Responsive 2D Materials Beyond Graphene. Adv. Funct. Mater. 2018, 28, doi:10.1002/adfm.201802500.
- Ye, ; Chao, D.; Shan, J.; Li, H.; Davey, K.; Qiao, S.-Z. Unveiling the Advances of 2D Materials for Li/Na-S Batteries Experimentally and Theoretically. Matter 2020, 2, 323–344, doi:10.1016/j.matt.2019.12.020.
- Walsh, A.; Hinkle, C.L. van der Waals epitaxy: 2D materials and topological insulators. Appl. Mater. Today 2017, 9, 504–515, doi:10.1016/j.apmt.2017.09.010.
- Zhao, ; Wan, J.; Yao, H.; Zhang, L.; Lin, K.; Wang, L.; Yang, N.; Liu, D.; Song, L.; Zhu, J.; et al. Few-layer graphdiyne doped with sp-hybridized nitrogen atoms at acetylenic sites for oxygen reduction electrocatalysis. Nat. Chem. 2018, 10, 924–931, doi:10.1038/s41557-018-0100-1.
- Qian, ; An, T.; Sarnello, E.; Liu, Z.; Li, T.; Zhao, D. Janus Electrocatalysts Containing MOF-Derived Carbon Networks and NiFe-LDH Nanoplates for Rechargeable Zinc–Air Batteries. ACS Appl. Energy Mater. 2019, 2, 1784–1792, doi:10.1021/acsaem.8b01923.
- Lim, ; Jin, X.; Jo, Y.K.; Lee, S.; Hwang, S.J. Kinetically Controlled Layer-by-Layer Stacking of Metal Oxide 2D Nanosheets. Angew. Chem. Int. Ed. Engl. 2017, 56, 7093–7096, doi:10.1002/anie.201701738.
- Xu, ; Huang, Z.; Wang, B.; Liang, Z.; Zhang, C.; Wang, Y.; Zhang, W.; Zheng, H.; Cao, R. A two-dimensional multi-shelled metal-organic framework and its derived bimetallic N-doped porous carbon for electrocatalytic oxygen reduction. Chem. Commun. 2019, 55, 14805–14808, doi:10.1039/c9cc08250f.
- Zhan, ; Chen, Z.; Zhang, Q. Recent progress in two-dimensional COFs for energy-related applications. J. Mater. Chem. A 2017, 5, 14463–14479, doi:10.1039/c7ta02105d.
- Wang, ; Yang, W.; Chen, X.; Wang, J.; Zhu, Y. Photocatalytic activity enhancement of core-shell structure g-C3N4@TiO2 via controlled ultrathin g-C3N4 layer. Appl. Catal. B Environ. 2018, 220, 337–347, doi:10.1016/j.apcatb.2017.08.004.
- He, ; Kim, C.; Lin, L.; Jeon, T.H.; Lin, S.; Wang, X.; Choi, W. Formation of heterostructures via direct growth CN on h-BN porous nanosheets for metal-free photocatalysis. Nano Energy 2017, 42, 58–68, doi:10.1016/j.nanoen.2017.10.043.
- Liu, ; Hu, K.; Yan, D.; Chen, R.; Zou, Y.; Liu, H.; Wang, S. Recent Advances on Black Phosphorus for Energy Storage, Catalysis, and Sensor Applications. Adv. Mater. 2018, 30, e1800295, doi:10.1002/adma.201800295.
- Zhang, Z.; El-Demellawi, J.K.; Jiang, Q.; Ge, G.; Liang, H.; Lee, K.; Dong, X.; Alshareef, H.N. MXene hydrogels: Fundamentals and applications. Chem. Soc. Rev. 2020, 49, 7229–7251, doi:10.1039/d0cs00022a.
- Han, ; Liu, H.; Chen, S.; Chen, Y.; Wang, S.; Li, Z. Fabrication and Characterization of MoS2/h-BN and WS2/h-BN Heterostructures. Micromachines 2020, 11, 1114, doi:10.3390/mi11121114.
- Wang, ; Zhang, L.; Huang, M.; Zhang, Q.; Zhao, X.; He, Y.; Lin, S.; Pan, J.; Zhu, H. One-step synthesis of a hierarchical self-supported WS2 film for efficient electrocatalytic hydrogen evolution. J. Mater. Chem. A 2019, 7, 22405–22411, doi:10.1039/c9ta07868a.
- Wang, ; He, H.; Pu, Z.; Chen, L.; Zhang, C.; Wang, Z.; Mu, S. Phosphorization engineering ameliorated the electrocatalytic activity for overall water splitting on Ni3S2 nanosheets. Dalton Trans. 2019, 48, 13466–13471, doi:10.1039/c9dt02841b.
- Canadell, ; LeBeuze, A.; El Khalifa, M.A.; Chevrel, R.; Whangbo, M.H. Origin of metal clustering in transition-metal chalcogenide layers MX2 (M = Nb, Ta, Mo, Re; X = S, Se). J. Am. Chem. Soc. 1989, 111, 3778–3782, doi:10.1021/ja00193a002.
- Chhowalla, ; Shin, H.S.; Eda, G.; Li, L.J.; Loh, K.P.; Zhang, H. The chemistry of two-dimensional layered transition metal dichalcogenide nanosheets. Nat. Chem. 2013, 5, 263–275, doi:10.1038/nchem.1589.
- Carroll, M.; Zhang, H.; Dunklin, J.R.; Miller, E.M.; Neale, N.R.; van de Lagemaat, J. Unique interfacial thermodynamics of few-layer 2D MoS2 for (photo)electrochemical catalysis. Energy Environ. Sci. 2019, 12, 1648–1656, doi:10.1039/c9ee00513g.
- Wang, ; Yan, M.; Zhao, K.; Liao, X.; Wang, P.; Pan, X.; Yang, W.; Mai, L. Field Effect Enhanced Hydrogen Evolution Reaction of MoS2 Nanosheets. Adv. Mater. 2017, 29, 1604464, doi:10.1002/adma.201604464.
- Masurkar, ; Thangavel, N.K.; Arava, L.M.R. CVD-Grown MoSe2 Nanoflowers with Dual Active Sites for Efficient Electrochemical Hydrogen Evolution Reaction. ACS Appl Mater Interfaces 2018, 10, 27771–27779, doi:10.1021/acsami.8b07489.
- Gopalakrishnan, ; Lee, A.; Thangavel, N.K.; Reddy Arava, L.M. Facile synthesis of electrocatalytically active NbS2 nanoflakes for an enhanced hydrogen evolution reaction (HER). Sustain. Energy Fuels 2018, 2, 96–102, doi:10.1039/c7se00376e.
- Mao, ; Wang, Y.; Zheng, Z.; Deng, D. The rise of two-dimensional MoS2 for catalysis. Front. Phys. 2018, 13, s11467–s018.
- Wei, ; Hsu, C.; Almakrami, H.; Lin, G.; Hu, J.; Jin, X.; Agar, E.; Liu, F. Ultra-high-aspect-ratio vertically aligned 2D MoS2-1D TiO2 nanobelt heterostructured forests for enhanced photoelectrochemical performance. Electrochim. Acta 2019, 316, 173–180, doi:10.1016/j.electacta.2019.04.090.
- Kim, ; Kang, D.; Lee, Y.; Hong, S.; Shin, H.G.; Bae, H.; Yi, Y.; Kim, K.; Im, S. 2D TMD Channel Transistors with ZnO Nanowire Gate for Extended Nonvolatile Memory Applications. Adv. Funct. Mater. 2020, 30, 2004140, doi:10.1002/adfm.202004140.
- Zhu, ; Mu, X.; van Aken, P.A.; Yu, Y.; Maier, J. Single-layered ultrasmall nanoplates of MoS2 embedded in carbon nanofibers with excellent electrochemical performance for lithium and sodium storage. Angew. Chem. Int. Ed. Engl. 2014, 53, 2152–2156, doi:10.1002/anie.201308354.
- Sun, ; Huang, D.; Wang, H.; Xu, G.-L.; Zhang, X.; Zhang, R.; Tang, Y.; Abd Ei-Hady, D.; Alshitari, W.; Saad Al-Bogami, A.; et al. 1T MoS2 nanosheets with extraordinary sodium storage properties via thermal-driven ion intercalation assisted exfoliation of bulky MoS2. Nano Energy 2019, 61, 361–369, doi:10.1016/j.nanoen.2019.04.063.
- Mahankali, ; Thangavel, N.K.; Gopchenko, D.; Arava, L.M.R. Atomically Engineered Transition Metal Dichalcogenides for Liquid Polysulfide Adsorption and Their Effective Conversion in Li-S Batteries. ACS Appl. Mater. Interfaces 2020, 12, 27112–27121, doi:10.1021/acsami.0c04281.
- Babu, ; Masurkar, N.; Al Salem, H.; Arava, L.M. Transition Metal Dichalcogenide Atomic Layers for Lithium Polysulfides Electrocatalysis. J. Am. Chem. Soc. 2017, 139, 171–178, doi:10.1021/jacs.6b08681.
- Thangavel, K.; Gopalakrishnan, D.; Arava, L.M.R. Understanding Heterogeneous Electrocatalysis of Lithium Polysulfide Redox on Pt and WS2 Surfaces. J. Phys. Chem. C 2017, 121, 12718–12725, doi:10.1021/acs.jpcc.7b01514.
- Radisavljevic, ; Radenovic, A.; Brivio, J.; Giacometti, V.; Kis, A. Single-layer MoS2 transistors. Nat. Nanotechnol. 2011, 6, 147–150, doi:10.1038/nnano.2010.279.
- Voshell, ; Terrones, M.; Rana, M. Thermal and Photo Sensing Capabilities of Mono- and Few-Layer Thick Transition Metal Dichalcogenides. Micromachines 2020, 11, 693, doi:10.3390/mi11070693.
- Masurkar, ; Thangavel, N.K.; Yurgelevic, S.; Varma, S.; Auner, G.W.; Reddy Arava, L.M. Reliable and highly sensitive biosensor from suspended MoS2 atomic layer on nano-gap electrodes. Biosens. Bioelectron. 2021, 172, 112724, doi:10.1016/j.bios.2020.112724.
- Coleman, N.; Lotya, M.; O’Neill, A.; Bergin, S.D.; King, P.J.; Khan, U.; Young, K.; Gaucher, A.; De, S.; Smith, R.J.; et al. Two-dimensional nanosheets produced by liquid exfoliation of layered materials. Science 2011, 331, 568–571, doi:10.1126/science.1194975.
- Jayabal, ; Saranya, G.; Wu, J.; Liu, Y.; Geng, D.; Meng, X. Understanding the high-electrocatalytic performance of two-dimensional MoS2 nanosheets and their composite materials. J. Mater. Chem. A 2017, 5, 24540–24563, doi:10.1039/c7ta08327k.
- Toh, J.; Sofer, Z.; Luxa, J.; Sedmidubsky, D.; Pumera, M. 3R phase of MoS2 and WS2 outperforms the corresponding 2H phase for hydrogen evolution. Chem. Commun. 2017, 53, 3054–3057, doi:10.1039/c6cc09952a.
- Huang, ; Feng, X.; Du, C.; Song, W. High-quality phosphorus-doped MoS2 ultrathin nanosheets with amenable ORR catalytic activity. Chem. Commun. 2015, 51, 7903–7906, doi:10.1039/c5cc01841b.
- Song, ; Park, C.; Choi, H.C. Synthesis and properties of molybdenum disulphide: From bulk to atomic layers. RSC Adv. 2015, 5, 7495–7514, doi:10.1039/c4ra11852a.
- Splendiani, ; Sun, L.; Zhang, Y.; Li, T.; Kim, J.; Chim, C.Y.; Galli, G.; Wang, F. Emerging photoluminescence in monolayer MoS2. Nano Lett. 2010, 10, 1271–1275, doi:10.1021/nl903868w.
- Tao, ; Gao, Y.; Talreja, N.; Guo, F.; Texter, J.; Yan, C.; Sun, Z. Two-dimensional nanosheets for electrocatalysis in energy generation and conversion. J. Mater. Chem. A 2017, 5, 7257–7284, doi:10.1039/c7ta00075h.
- Tsai, ; Chan, K.; Nørskov, J.K.; Abild-Pedersen, F. Rational design of MoS2 catalysts: Tuning the structure and activity via transition metal doping. Catal. Sci. Technol. 2015, 5, 246–253, doi:10.1039/c4cy01162g.
- Huang, ; Feng, X.; Du, C.; Wu, S.; Song, W. Incorporated oxygen in MoS2 ultrathin nanosheets for efficient ORR catalysis. J. Mater. Chem. A 2015, 3, 16050–16056, doi:10.1039/c5ta01600b.
- Chen, ; Liu, T.; Wang, P.; Zhao, J.; Zhang, C.; Cheng, R.; Li, W.; Ji, P.; Pu, Z.; Mu, S. Ionothermal Route to Phase-Pure RuB2 Catalysts for Efficient Oxygen Evolution and Water Splitting in Acidic Media. ACS Energy Lett. 2020, 5, 2909–2915, doi:10.1021/acsenergylett.0c01384.
- Tang, ; Meng, X.; Deng, D.; Bao, X. Confinement Catalysis with 2D Materials for Energy Conversion. Adv. Mater. 2019, 31, e1901996, doi:10.1002/adma.201901996.
This entry is adapted from the peer-reviewed paper 10.3390/mi12030240