An efficient carbon dioxide reduction reaction (CO2RR), which reduces CO2 to low-carbon fuels and high-value chemicals, is a promising approach for realizing the goal of carbon neutrality, for which effective but low-cost catalysts are critically important. Many inorganic perovskite-based materials with tunable chemical compositions have been applied in the electrochemical CO2RR, which exhibited advanced catalytic performance.
1. Introduction
The booming development of industry and the economic level of modern society lead to an exponential growth in demand for energy, while the massive consumption of fossil fuels has resulted in some terrible environmental problems [
1,
2]. According to a report by the International Energy Agency (IEA), the total global greenhouse gas emissions in 2021 reached an equivalent of 40.8 billion tons of carbon dioxide (CO
2), in which fuel combustion and industrial production accounted for ~89% [
3,
4]. Several strategies and policies such as the Paris Agreement [
5,
6] and China’s “3060 two carbon goals” have been proposed to tackle carbon emission issues, which have stimulated extensive fundamental studies on developing efficient materials and technologies to convert CO
2 into value-added chemicals.
The electrochemical reduction in CO
2, activated by electricity from renewable energy sources such as wind, solar, etc., is one of the most promising methods for realizing the re-utilization of CO
2 and dealing with the intermittency problem of renewable energy sources [
7,
8]. However, due to the stable chemical properties of CO
2 molecules, the CO
2 reduction process usually requires relatively harsh operation conditions, and the reaction pathways are greatly complicated, leading to varieties of products (e.g., CO, CH
4, CH
3OH, HCOOH, C
2H
4, C
2H
6, CH
3CH
2OH, CH
3COOH, CH
3CH
2CH
2OH, etc.) [
9], despite the distinct equilibrium potentials of producing these chemicals (
Table 1) [
10]. Therefore, efficient catalysts with high activity, target product selectivity, and long-term stability are required to facilitate the CO
2 reduction reaction (CO
2RR) to meet the requirements of practical applications. Many significant achievements concerning noble-metal free electrocatalysts, especially inorganic perovskite oxides, which have some promising compositional and structural characters, have recently been obtained.
Table 1. Electrochemical reactions in CO
2RR under equilibrium potentials [
11].
2. Fundamentals of CO2RR
A CO
2 reduction reaction (CO
2RR) occurs at the interface of the tri-phase (CO
2 gas, liquid electrolyte, and solid catalyst surface), involving multiple electron and proton transfer processes. Generally, three steps are required for CO
2RR, as shown in
Figure 1 [
8,
12,
13]: (1) the CO
2 molecules are adsorbed on the active sites on the catalyst surface; (2) the adsorbed CO
2 molecules transform to a CO
2•− intermediate, and this initial electron transfer step is usually deemed the rate-determining step (RDS) of CO
2RR; and (3) the generated products are desorbed and depart from the catalyst surface, making the active sites ready for the subsequent catalytic cycle. Nonetheless, the detailed reaction mechanisms are far more complicated than the abovementioned three basic steps, and the reaction pathways are distinct for different catalyst materials, even for the same product [
14].
Figure 1. Three main steps on the catalyst surface of CO2RR processes. Black spheres represent carbon atoms; red spheres represent oxygen atoms; white spheres represent hydrogen atoms; orange atoms represent the CO2RR catalyst.
Laboratory-scale CO
2RR is usually conducted in a three-electrode system, which is also the most widely used configuration in studies that investigate the catalytic performance of perovskite-based electrocatalysts. The system consists of a cathode (working electrode, where CO
2RR occurs), an anode (usually platinum or nickel materials), and a reference electrode (Hg/HgO in an alkaline electrolyte, Ag/AgCl in a neutral or acidic electrolyte). Under the negative working potentials, CO
2RR and the oxygen evolution reaction (OER) occur over the cathode and anode, respectively. Studies of CO
2RR generally focus on the cathode, including both the catalytic materials and electrode structure. Herein, we only focus on the development of electrocatalysts for CO
2RR. Generally, compared with metal catalysts, metal oxides have flexible and tunable structures, as well as interesting p–d coupling effects, which provide more abundant opportunities for tuning the electronic configuration of transition metal-based active sites and hence lead to promising catalytic properties. Among all the CO
2RR catalysts that have been explored to date, perovskite-based materials exhibit some interesting characteristics and great potential in various electrochemical reactions [
15,
16,
17,
18,
19,
20].
3. Fundamentals of Perovskite Oxides
Perovskite is a family of compounds that have a general chemical formula of ABX
3, the A-site elements could be rare-earth, alkaline-earth, or alkaline metals (e.g., Sr, La, Pr, Nd, Li, Na, Mg, Ca, etc.), while the B-site elements are transition metals (e.g., Ti, V, Mn, Fe, Co, Ni, Cu, etc.) that usually work as the active components during various catalytic reactions due to the existence of abundant d electrons and unfilled d orbitals. The anion X is usually oxygen for most of the perovskite-based CO
2RR catalysts that will be discussed in this review article. Arising from the distinct atomic features of different A and B elements, the crystal structure of perovskites could be tuned by modulating the chemical compositions, which range from cubic to orthorhombic, tetragonal, or trigonal. In 1926, Goldschmidt proposed a relationship between the bond length of both A-X and B-X bonds to predict if a combination of a pair of cations could form the perovskite structure [
21]. Then, this theory was optimized to use ionic radii as a substitute for bond length in order to expand the application range. The equation of modified Goldschmidt’s rule is displayed as follows:
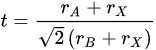
where
t is called the tolerance factor,
rA is the radius of the A-site cation,
rB is the radius of the B-site cation, and
rX is the radius of the anion. The compound could be in an ideal perovskite structure when the tolerance factor is equal to precisely 1. Generally, the perovskite structure is stable when the tolerance factor is in the range of 0.75 to 1.00 [
22]. If
t lies in the approximately range of 0.9 to 1.0, the perovskite tends to exist in the ideal cubic structure. When
t is lower than 0.9 but higher than 0.71, the octahedral structure will distort to break the cuboctahedral coordination and form a structure with a lower symmetry than the cubic one.
In addition to the simple ABX
3 type, the family of perovskite materials also contains many other derivatives that show different atomic ratios, such as the Ruddlesden–Popper perovskite (RP-type) [
22], Dion-Jacobson perovskite [
23], Aurivillius perovskite [
24], etc. All these perovskite derivatives can be realized by adjusting the thickness of perovskite units or inter-layer species. Regular and repeated phases can be synthesized when the structures are ordered, while disordered modules are non-stoichiometric. Such perovskites can be regarded as “layered materials”, which leads to their special physical structures and chemical characterizations. What is more, the special crystal structure of perovskites allows some elements to exist in unusual or mixed valence states with non-stoichiometric ratios of oxygen, leading to tunable and unique chemical properties that would further benefit the catalytic reactions. Actually, the positive roles of oxygen vacancies in perovskites for catalysis have been extensively investigated. It has been found that the moderate concentration of oxygen vacancies on the catalyst surface could effectively suppress the competitive hydrogen evolution reaction (HER) and also facilitate charge transfer during the CO
2RR process [
25].
The electrical conductivity of perovskites is another significant property when applied as an electrocatalyst. The RP-type perovskite materials were found to have high electrical conductivity due to the BO
6 octahedra structure [
26], which benefits the charge transfer process during electrochemical reactions [
27]. Compared with the simple ABO
3 perovskite, the RP-type perovskite (A
2BO
4) shows better electrical conductivity; moreover, the capability can be improved with the increasing n number because of the higher three-dimensional layered structure. In addition, the RP-type perovskite materials are stable enough under different pH conditions and oxidative potentials [
28], making them promising electrocatalysts for many energy conversion reactions. In summary, the unique physicochemical properties of perovskite materials endow them with a broad application in various fields, such as energy conversion, storage, and electrochemical sensors [
29,
30].
4. Fabrication Methods of Perovskite Materials
In addition to chemical composition and crystal structure, particle size and microstructure also affect the performance of catalysts. Consequently, many approaches have been developed to synthesize perovskites with controlled particle size and morphology, such as wet chemistry methods, deposition-based approaches, templated-assisted synthesis, electrospinning, infiltration, exsolution, etc. In wet chemical synthesis, sol-gel processes are widely used to prepare nanosized perovskites [
31,
32]. In this method, citric acid [
33] and ethylenediamine tetra-acetic acid [
34] are often applied as the complexing agents to coordinate with the metal ions. A subsequent high-temperature calcination process should be carried out to remove the organics and facilitate the growth of perovskite crystals. Although the sol-gel method can produce purer-phase perovskites with higher surface areas when compared with the conventional solid-state method, the high-temperature calcination would inevitably lead to large agglomerated particles with a size of tens to hundreds of nanometers [
35]. Template-assisted approaches have also been developed to synthesize perovskites with a porous microstructure [
36]. Generally, a porous soft or hard template will be introduced in the preparation procedures, and then removed via calcination or chemical etching. The soft templates usually are self-assembled amphiphilic molecules [
37,
38] such as surfactants, and the hard ones can be silica-based molecular sieves [
39,
40] and organic polymethyl methacrylate (PMMA) [
41,
42]. The choice of templates should be based on the requirements for the pore size of the targeted structure of perovskites. Until now, perovskites with micropores, mesopores, three-dimensional ordered macropores, and hierarchical pores have been successfully prepared [
43,
44]. The porous microstructure could not only increase the surface area and hence provide more active sites but also provide convenient mass transfer channels, contributing to the greatly improved catalytic performance of perovskite-based materials for CO
2RR.
This entry is adapted from the peer-reviewed paper 10.3390/molecules28248154