1. Introduction
Eugenol (4-allyl-2-methoxyphenol, EUG) (Scheme 1), a volatile naturally occurring phenol, is present in extracts of many botanical species. EUG has been identified in essential oils of cinnamon, bay and tulsi leaves, turmeric, nutmeg, and the most eugenol-rich clove
Syzygium aromaticum (45–90% of 4-allyl-2-methoxyphenol)
[1][2]. The antimicrobial, antifungal, antioxidant, anticancer, anti-inflammatory, analgesic, repellent, and insecticidal activities of this compound are well known. Because of those beneficial features, eugenol has been employed in food processing and biomedical industries and in a wide range of pharmaceutical, cosmetic, and dental care applications
[1].
Scheme 1. Chemical structure of 4-allyl-2-methoxyphenol.
Bioactive compounds with antimicrobial activity, such as EUG, can be an alternative to antibiotics against many microbial pathogens, and thus reduce the increasing resistance of microorganisms to antibiotics. The minimum inhibitory concentration (MIC) and minimum bactericidal concentration (MBC) of eugenol against selected pathogenic bacteria were found to be, respectively, 0.0312–8 μg/mL and 0.0625–16 μg/mL
[3]. Eugenol showed significant antimicrobial activity against
Escherichia coli (MIC = 0.125 μg/mL; MBC = 0.250 μg/mL). The time kill curve revealed a rapid reduction in
E. coli to undetectable levels in the presence of EUG. It was postulated that the compound alters cell membrane permeability, leading to the leakage of intracellular contents. The disruptive action of EUG on the cytoplasmic membrane was demonstrated by measurements of intracellular ATP. The MIC value of eugenol was enhanced in the presence of divalent cations, and an increase in the bacterium membrane depolarization was observed. Microscopic studies proved morphological and physiological changes in
E. coli caused by 4-allyl-2-methoxyphenol. After membrane disruption, eugenol may further interact with the intracellular organelles causing complete damage to bacterial cells (Scheme 2).
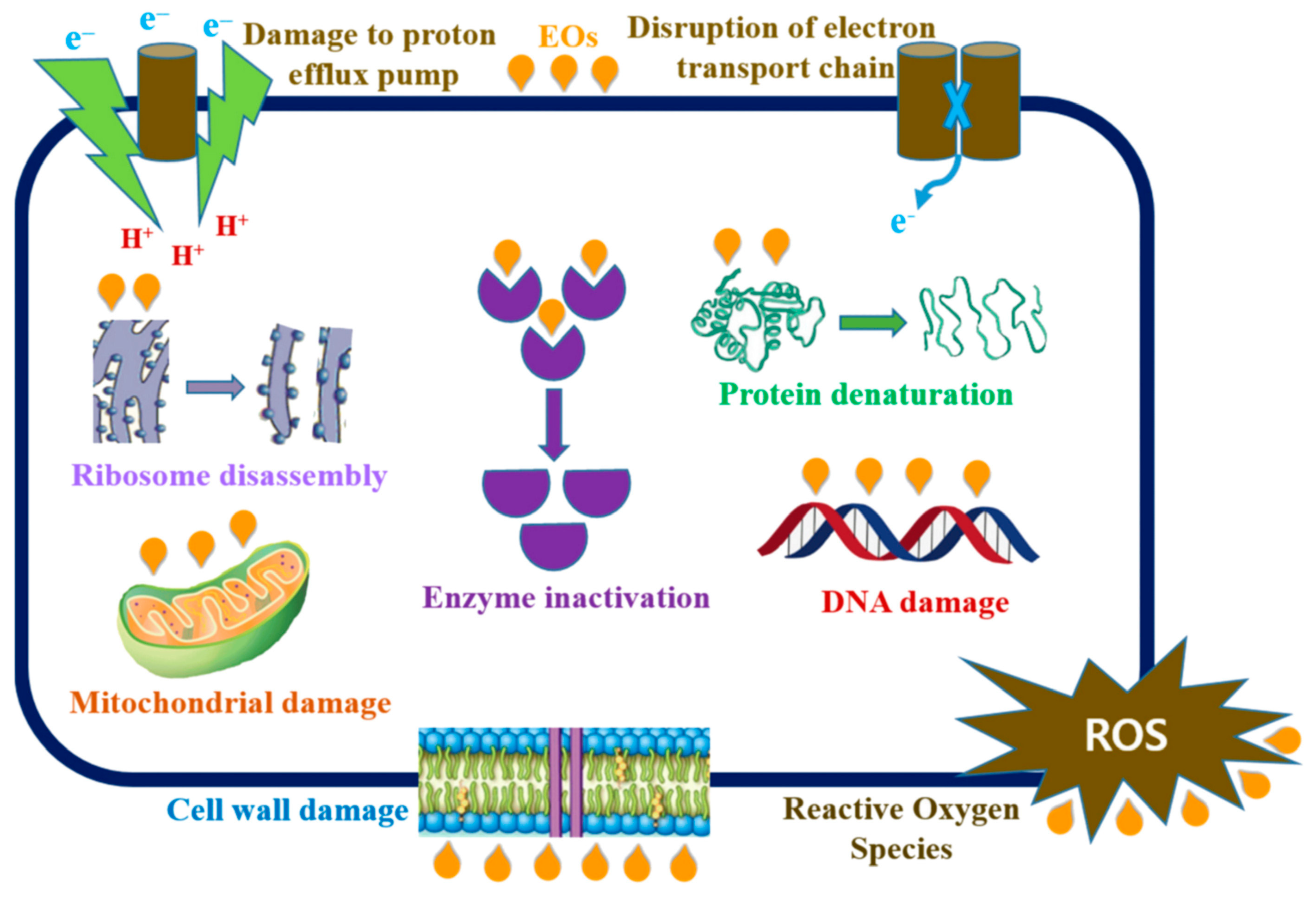
Scheme 2. Postulated mechanism of intracellular action of essential oils on bacterial cells
[4].
Interestingly, the effectiveness of antimicrobials (including eugenol) depends not only on their overall concentration but also on the time used to reach that concentration. Some microorganisms may become more tolerant when treated with lower concentrations of EUG. This may be particularly important for encapsulated antimicrobial systems, where the active substances are released gradually and their minimal lethal concentration (MLC) levels are only reached after a certain period of time. Adaptation to the environmental stressor involves membrane composition changes, which make the microbial cells more resistant.
The time between antimicrobial sublethal doses was shown to have a significant effect on the efficacy population dynamics of
Staphylococcus carnosus,
Listeria innocua,
Escherichia coli K12, and
Pseudomonas fluorescens exposed to MLC of eugenol (initially, or to half doses over time)
[5]. As expected, complete cell reduction occurred after immediate exposure to the full MLC dose, regardless of the type of microorganisms. The high antimicrobial efficacy of EUG on
E. coli K12 was independent of the timing of the two doses. For other tested strains, the effect of sequential dosing varied significantly. The effectiveness of EUG against
S. carnosus and
L. innocua was lower the later the second half dose was applied. In the case of
P. fluorescens, a single MLC dose of EUG reduced the number of cells below the detection level, while the sequential application of two half doses had only a bacteriostatic effect. Less severely stressed microbial populations developed resistance to EUG. To minimize such an adaptation, two consecutive half-doses should be applied fairly quickly, and exposure to low levels of stress should be avoided.
The application of several different stressors (e.g., two different antimicrobials
[6] or an antimicrobial and high/low temperature
[7]) simultaneously or sequentially may be a solution to avoid the phenomenon of acquired resistance. For example, the application of two antimicrobials, eugenol and lauric arginate (LAE), against
S. carnosus,
L. innocua,
E. coli K12, and
P. fluorescens was most effective if they were added simultaneously at MLC and at the beginning of the incubation period
[6]. The treatment with first eugenol and then LAE at half of their MLC was efficient against
S. carnosus and
P. fluorescens but no sequence effects were observed against
L. innocua and
E. coli K12. These results were attributed to the adaptation of cells after treatment with the first active agent, which provided better protection against the second antimicrobial.
2. Eugenol-Based Polymers and Composites for High-Performance Antimicrobial Coatings
Polymers obtained with the use of eugenol are receiving growing interest because of their sustainability and enhanced physicochemical characteristics. The development of such polymeric materials designed to control bacterial biofilm growth is an important practical approach. They can be used to generate bacteria-repellent or antibacterial coatings aimed at preventing bacterial colonization. EUG-derived polymers can be used as coatings in a wide range of applications
[8]. Excellent thermosetting materials were also obtained with monomers that are derivatives of eugenol
[9][10]. For example, eugenol molecules were transformed into 4,4′-(butane-1,4-diyl)bis(2-methoxyphenol) by the Ru-catalyzed olefin metathesis coupling reaction
[11]. The product was then converted into a polycyanurate thermoset resin that exhibited a glass transition temperature (T
g) of 186 °C and was thermally stable above 350 °C. The water uptake of the cured material was only 1.8% after immersion in hot water (85 °C) for four days. The high hydrolytic stability makes this polymer well-suited even for maritime environments. EUG molecules can also be transformed into other monomers suitable for the free radical polymerization reaction, including EUMA—eugenyl methacrylate, and thus particles of poly(DMA-co-EUMA) (DMA—dopamine methacrylamide) were obtained via precipitation copolymerization
[12]. The antibacterial activity test against
E. coli showed a >90% antibacterial rate of PD5E5 and PD3E7 particles (5:5 and 3:7 molar ratios of DMA to EUMA). Another derivative of eugenol—4-allylpyrocatechol—was used for the preparation of photocurable polymer networks of high macroscopic adhesion to glass, marble, aluminum, and steel
[13]. Eugenol was also used for the synthesis of environmentally sustainable polybenzoxazines
[14], polybenzoxazine-POSS nanocomposites
[15], and analogues of urushiol
[16] of superior adhesion, mechanical strength, and antioxidant properties. These polymerization processes are green and do not involve the evolution of volatiles.
Eugenol-derived polymers are also capable of self-healing, which is a very important feature regarding the stability of coating materials that affect their antimicrobial/antibiofilm performance. The process can be based either on thiol-oxidation reactions
[17][18][19] or hydrogen bonding
[20]. Interestingly, eugenol derivatives can take part in a self-healing process through mechanisms based on the formation of multiphase structures in polymer composites. A biobased molecular glass (ET-eugenol) obtained by thermochemical conversion of epoxidated eugenol and then incorporated into the matrix of polymerized soybean oil (p-ESO) can be an example
[21]. The scratch damage on the surface of ET-eugenol/p-ESO (1:2 wt./wt.) composite was healed at 90 °C within 15 min or by UV radiation within 20″ effectively (efficiency up to 88%) and without a dimension change. Furthermore, the incorporation of ET-eugenol improved the mechanical properties of the p-ESO matrix as comparative tensile tests of these materials showed a 2.7-fold increase in ultimate stress.
The synthesis reactions of some EUG-based polymers involved phenolic groups, which may inevitably lead to a partial loss of the antioxidant activity of eugenol. Nevertheless, several systems of this kind showed significant antimicrobial activity. For example, water-insoluble polyeugenol of a high molecular mass (~10 kg mol
−1, DP = 60) was synthesized by cationic polymerization and showed antibacterial activity with a slow response against
E. coli and
S. aureus [22]. Polyeugenol of a high molecular weight (~800–2200 kg mol
−1) was also tested against
S. aureus and
E. coli [23]. The antibacterial action was evaluated with the well diffusion method at 1, 2, 3, 4, and 5% concentrations to observe inhibition zones of 17.42, 17.76, 18.79, 21.42, and 22.55 mm for
S. aureus and 15.87, 17.23, 17.56, 18.24, and 19.21 mm for
E. coli. These results indicate that antibacterial activity was rather strong. It was supported by the strong antioxidant activity of the polymer indicated in the tests against free radical DPPH (2,2-diphenyl-1-pycrylhydrazyl), which resulted in an IC
50 value of 80.47 µg/mL.
Eugenol-derived polymeric films (EDF) can be deposited on stainless-steel surfaces using atmospheric pressure plasma discharge
[21]. Scanning electron microscopy showed that the entire surface of the substrate was covered with circular structures of ~10–20 µm in diameter. FT-IR spectra showed that the hydroxyl and aromatic groups, which are key features for the antibacterial activity of native eugenol, were preserved. An increase in the hydrophilicity of the surface after the deposition was revealed. EDF deposits can be potentially of use in coating processes for biocompatible materials. The adhesion and proliferation of
E. coli and
S. aureus on EDF surfaces were inhibited by more than 78 and 65%, respectively, in comparison with control polystyrene plates.
Eugenol was also used for the chemical modification of inorganic and organic polymeric materials. Hydrosilylation of the alkyl group in eugenol with hydrogen-containing MQ silicone resin (M: monofunctional and Q: tetrafunctional siloxane units) resulted in a hybrid bio-phenol MQ silicone resin (BPMQ)
[24]. The thermal stability of BPMQ was significantly improved. The maximum degradation rate increased from 250 °C (MQ precursor) to 422.5 °C (BPMQ) due to the modification with EUG. The mass of residual yields left at 600 °C increased accordingly, from 2.0% (MQ) to 28.3% (BMQ). The resin was also investigated for its antibacterial properties against
E. coli, markedly enhanced as a result of EUG grafting (
Figure 1). Hybrid materials of this type can be used for biomedical silicone rubber products or pressure-sensitive adhesives.
Figure 1. Antimicrobial activity presented by changes in agar plate cultures: The blank group (
a), HMQ (
b), eugenol (
c), and BPMQ (
d)
[24].
Essential oils (EO), such as eugenol, can be also physically blended with various polymers. The structural stability of such materials and their antimicrobial performance are limited by the phase separation phenomena, especially if there are no specific interactions between EUG and the polymer matrix. For example, poly(ethylene-co-vinyl acetate) copolymer (EVA) films containing citronellol, eugenol, and linalool were prepared and evaluated for their antimicrobial and antibiofilm action against
Listeria monocytogenes,
S. aureus,
Staphylococcus epidermidis,
E. coli, and
P. aeruginosa (mono- and dual-species tests)
[25]. The addition of the essential oils influenced the mechanical properties of EVA (15% elastic modulus decrease, 30% tensile strength decrease, 10% elongation at break increase). EVA/citronellol and EVA/eugenol at 7 wt% of the essential oils content showed the best inhibition of bacteria growth (30–60% and 15–30%, respectively, after 24–48 h of incubation). However, in both cases, the inhibition decreased after 240 h of incubation. Antibiofilm action of the composites was strong, even after prolonged incubation time. A 40–70% decrease in the bacterial biomass of
L. monocytogenes,
S. aureus, and
E. coli was found for the EVA/EO composites in comparison with the pure EVA control. Interestingly, better results were achieved with EVA/eugenol than EVA/citronellol when inoculated simultaneously with combinations of
S. aureus and
E. coli. The biomass accumulated was higher (EVA + citronellol) or lower (EVA + eugenol) than that in monoculture biofilms. Similar findings were obtained with measurements of the metabolic activity of viable cells with 2,3-bis [2-methyloxy-4-nitro-5-sulfophenyl]-2H-tetrazolium-5-carboxanilide assay.
Eugenol was also grafted to the copolymer of carboxymethyl cellulose (CMC) and cysteamine hydrochloride (CYST) by the thiol-ene click reaction between the allyl group of EUG and mercapto-groups of CYST
[26]. The resulting amphiphilic polymer (CMC-CYST-EUG) showed a 7.8-times longer duration of antimicrobial activity compared to pure EUG under the same environmental conditions. Cytotoxicity tests show that the polymer is safe at low concentrations. CMC-CYST-EUG can also increase the shelf life of fruits and vegetables owing to their good adhesion. The efficacy of CMC-CYST-EUG in pest control was significantly higher than that of EUG.
In summary, inorganic and organic macromolecular systems functionalized with eugenol, both as a building block and as a doping additive, show interesting physicochemical, mechanical, and antimicrobial properties. This approach increases the potential contact time of eugenol with pathogenic micro-organisms and increases the efficiency of their inactivation. Of particular interest is the possibility of using eugenol for antimicrobial modification of ‘green’ biopolymers.
3. Eugenol-Based Materials for Food Packaging
Bioactive and biodegradable hybrid materials are a very interesting solution for, e.g., environmentally friendly food storage
[4][27][28]. Packaging technologies have to meet growing demands regarding the extended shelf-life and good quality of products, especially fresh or low-processing food. The improvement of food preservation via the design of new technologies incites growing interest in biocompatible and preferably biodegradable antimicrobial/antibiofilm materials. The process of making “green” polymeric materials for active packaging is related both to the type of matrix and the incorporated additives.
It was shown in the tests performed with selected bacterial strains isolated from dairy products (
E. coli,
S. aureus;
Bacillus pumilus,
Bacillus subtilis,
Bacillus tequilensis, and
Stenotrophomonas maltophilia) that biodegradable polymer films of poly(lactic acid) (PLA), poly(butylene adipate-co-terephthalate) (PBAT), and poly(butylene succinate) (PBS) have a varying propensity for biofilm growth
[29]. Neat PLA was less prone to biofilm settlement than PBS. The antimicrobial activity and the reduction in biofilm formation on polymer films were improved upon the inclusion of thymol and eugenol into these materials. Biofilm formation was not detected on polymer films with incorporated antimicrobial compounds.
On the other hand, polylactide-based materials covalently grafted with eugenol did not exhibit high antimicrobial action
[30]. The polymers were synthesized by homopolymerization reactions of eugenol-bearing L-malic acid-derived O-carboxyanhydride (L-MalOCA) or its copolymerization at two different ratios with the L-lactic acid-derived OCA (L-LacOCA) monomer. The thermal properties of the resulting polymers depended on the content of L-MalOCA. The antimicrobial activity of the hybrid materials against common food-related bacteria, namely
E. coli,
Pseudomonas aeruginosa,
S. aureus, and
B. cereus, was tested. The action of the eugenol-rich poly(L-MalOCA) product was detected against
S. aureus and
B. cereus, and to a minor extent, against
P. aeruginosa. No inhibition was found in tests against
E. coli.
Eugenol, cinnamaldehyde (CIN), and carvacrol (CAR) were added to enhance the antimicrobial properties of zein-based materials
[31]. Polyethylene glycol (PEG) and oleic acid (OA) were used as plasticizers to improve the mechanical properties of zein. Compared to OA, PEG efficiently improved the tensile strength and elongation (%E) of zein films but induced weaker water barrier properties. The EO-embedded zein films demonstrate a gradual release of EO (up to 96 h) and showed even better antimicrobial effects against
S. aureus and
E. coli than neat EO in a filter paper sample (FPS). This can be explained by the difference in release rates for EO in zein-PEG and FPS. The chemical associations lead to a controlled release of EO. EO interacts with zein through weak chemical bonds and their molecules are chemically entrapped in the zein film, thus the evaporation rate is low. The strongest antimicrobial effect was noted for CIN (16.67 mm inhibition zone against
S. aureus for zein/PEG with 5% CIN and 4.67 mm for pure CIN). CAR was active only at a concentration of 3% in zein-PEG, and it was comparably effective toward both bacteria. Unfortunately, EUG showed no inhibitory effect against
S. aureus and
E. coli at the 1% and 3% concentration levels, respectively. Tests at 8% of EUG indicated that the G- bacterium
E. coli was more sensitive to EUG than G+
S. aureus, either entrapped in zein-PEG or in pure form in FPS.
Eugenol was also added to improve the antimicrobial properties of a blend of PLA and zein of improved mechanical and barrier properties (elongation, tensile strength, water vapor, and gas permeabilities)
[32]. EUG significantly inhibited the growth of both
S. aureus and
E. coli. The migration tests of eugenol from the polymer composites into distilled water and three food simulants (10% (
v/
v) ethanol simulating hydrophilic food, 50% (
v/
v) ethanol for lipophilic products, and 3.0% (
w/
v) acetic acid solution for acidic hydrophilic products with pH < 4.5) were conducted. Interestingly, the results illustrated the different actions of various types of food on the migration rates of eugenol. The EUG migration rate was controlled by zein. In the case of hydrophilic food, it was generally faster for materials with a higher content of PLA, and transport of EUG in this case was slowed down by zein nanoparticles. EUG molecules moved most rapidly in the environment simulating lipophilic products. In such systems, hydrophobic interactions of both zein and eugenol with the stimulant exist. The migration of lactic acid (LA), that is the degradation product of PLA, to water and three different food simulants depended on the content of PLA, but the amount was generally low (<2.5 mg/dm
2 in 5 days). This demonstrates the lack of degradation of the polylactide by eugenol.
Gelatine (GL)/chitosan (Ch)-based active, biodegradable, and edible films and coatings containing eugenol and oregano (
Origanum vulgare) essential oil (OEO) in different OEO:EUG ratios were designed for food preservation
[33]. Retardation of the oxidative processes and the growth of pathogenic microorganisms (
S. aureus and
E. coli) on the surface of food (e.g., fresh cheese) could be achieved. The optimal activity of systems containing 0.50% EUG + 0.50% OEO and 0.25% EUG + 0.75% OEO is attributable to the synergistic antimicrobial effect of EUG, OEO, and Ch. The water vapor barrier properties in OEO-containing films were markedly enhanced despite a slight phase separation in the hybrid materials, especially with the high OEO:EUG ratio. The observed higher moisture content and water solubility values can be related to the morphology of those coatings. The optimal film structure (a sample with 0.5% wt/wt of OEO + 0.5% wt/wt of EUG content) correlated with preservation properties of coatings that were tested on fresh cheese during 14 days of storage at 4 °C. However, none of the tested coatings prevented WL (freshness) in the cheese samples.
The eugenol content of physical blends with biocompatible polymers is not high since larger amounts of EUG cannot be retained by the polymer matrix. It is thus desirable to introduce an ingredient that traps the eugenol molecules. For example, eugenol can form inclusion complexes with β-cyclodextrins (βCD). The formation of host-guest interactions was exploited for the preparation of a water-absorbent antibacterial mat, made of electrospun fibers composed of polylactic acid-β-cyclodextrin/eugenol (PLA-β-CD/EUG), a super absorbent polymer (SAP), and filter paper
[34]. The mats were shown to restrain the growth of
S. aureus,
E. coli,
P. aeruginosa,
L. monocytogenes,
Bacillus pyocyaneus, and
Salmonella typhimurium. Comparative EUG release tests from PLA-β-CD/EUG and PLA-EUG fiber membranes demonstrated that the maximum release time for the latter was 4-fold lower. Stable release profiles were then observed for both samples over a period of up to 40 min. The slower and steady EUG release profile of PLA-β-CD/EUG fiber membranes can be attributed to host–guest interactions between EUG and β-CD. In addition to the demonstrated inhibitory effect on both G+ and G- bacteria, the water-absorbent antibacterial PLA-β-CD/EUG mats had a longer-lasting EUG release effect in different food simulants. The slowest eugenol delivery was found for oily food, which may be promising in meat preservation.
Fish gelatine mats containing cinnamaldehyde (CEO), limonene (LEO), and eugenol at 1, 3, and 5% with β-cyclodextrins at an equimolar ratio were prepared from electrospun fibers
[35]. It was expected that the diverse structure of EO molecules (aldehyde, terpene, and phenol derivatives) would cause different interactions with βCD. The presence of essential oils did not disturb the morphology of fibers (defect-free) but reduced their mechanical properties (tensile strength and elongation at break), despite the improvement in the gelatine melting temperature by 3 to 13 °C. Although all three types of gelatine mats showed similar antifungal activity at 5% concentration of essential oils, their antibacterial properties depended on the type of EO additive. The highest radical scavenging activity (96.5%) was obtained with EUG, while cinamaldehyde resulted in the highest inhibition zone (27.73 mm). The functional stability of the developed mats and a slow release of EOs for 60 days at ambient temperature were noted, which indicates the applicative potential as active antimicrobial packaging for low-to-intermediate moisture foods.
Packaging materials with EUG molecules built into the macromolecular system were also obtained. Polymacrolactones with pendant eugenol moieties were prepared via the functionalization of poly(6HDL-
co-PDL) copolymers of random structure that were obtained in ring-opening copolymerization of ω-6-hexadecenlactone (6HDL) and ω-pentadecalactone (PDL) carried out in the presence of a pyridylamidozinc(II) complex
[36]. Eugenol was converted into 4-(3-mercaptopropyl)-2-methoxyphenol (TE) and then grafted by thiol-ene addition to the unsaturated C=C bonds of 6HDL units in poly(6HDL-co-PDL). The structure and thermal properties of the final products were modulated by the amount of eugenol-derived side groups. The antimicrobial activity of TE-functionalized copolymers against
E. coli was also dependent on the content of TE units in the films.
Copolymers of eugenol-trithiol methylenebisacrylamide (E3TMBA) and eugenol-trithiol, allyl eugenol (E3TAE) of high thermal stability (5.0% mass loss at 179 °C for E3TMBA and 255.9 °C for E3TAE) were synthesized via solvent-free photopolymerization of eugenol and trithiol with methylenebisacrylamide (MBA) and allyl eugenol (AE) as crosslinkers
[37]. E3TMBA and E3TAE were moderately hydrophilic (contact angle values (θ) of 74° and 58.4°, respectively). They exhibited glass transitions at 48 °C and 30 °C, respectively. The trend correlated with their mechanical properties (tensile strength of 0.463 MPa and a 6% elongation recorded for E3TMBA and 0.378 MPa/33% for E3TAE). The antibacterial activity against
E. coli (12/24 h inhibition values: 46.65%/77.04% (E3TMBA) and 55.7%/83.04% (E3TAE)) and
S. aureus (12/24 h inhibition values: 64.11%/82.06% (E3TMBA) and 68.8%/80.04% (E3TAE)) was good, which is promising for their food packaging potential.
Eugenol and three dithiols of various structures were used for the preparation of macromolecular antioxidants (MAOs), subsequently applied as additives to poly(vinyl alcohol) (PVA)
[38]. The polymer main-chain morphology and properties were tuned by the structure of dithiols. Mono-phenolic and catechol moieties from EUG were distributed along the polymer backbone, with coexisting thioethers, oxyethers, or vicinal diol groups. Macromolecules with catechol groups and vicinal diols exhibited a synergistic effect to enhance the anti-oxidation storage stability. Solution-cast hybrid films exhibited enhanced mechanical strength and toughness. Excellent scavenging of DPPH radicals was noted for PVA containing ≥5% MAO (at 7.5% MAO complete scavenging after 3 min).
For packaging applications, eugenol can be also used in the neat form, for physical modification of polymeric materials. Their final properties depend on the processing conditions. For example, EUG was added to linear low-density polyethylene (LLDPE) films under conditions of supercritical CO
2-assisted impregnation
[27]. The effect of this process can be modulated by the pressure and depressurization rate. Binary mixtures of EO containing thymol, carvacrol, citral, and eugenol were also introduced into polypropylene (PP)/polyamide (PA)/nanoclays composite blends processed into thin films
[39]. Their release kinetics were studied in correlation with their capacity to inhibit the growth of
E. coli and fungi over time. The inhibition of fungal growth on food samples for over 50 days indicated the potential use of the developed EO-loaded films as active food packaging.
4. Encapsulation of Eugenol in Polymeric Materials for Biomedical Applications
Increasing antibiotic resistance of bacterial strains necessitates the development of new antibacterial agents and therapies to prevent uncontrolled disease-causing pathogenic microorganisms. The increasing resistance of bacterial strains to antibiotics requires the development of new antimicrobial agents and therapies to prevent pathogenic microorganisms from causing disease uncontrollably.
Nano-based and combinatorial strategies offer solutions for reducing the use of antibiotics. The toxicity of various inorganic and organic antibacterial compounds has caused increasing interest in medicinal plant extracts with biocompatible and potent antibacterial characteristics. Such green antimicrobials are volatile and most often insoluble in water; thus, their bioavailability is rather poor. Nano-scale technologies provide a range of solutions to the problem of their poor bioavailability as well as oxygen sensitivity. As an additional result, the therapeutic potential of the active compounds may be enhanced
[40][41][42][43][44]. Bioactive phytochemicals can be encapsulated or entrapped within inorganic or organic hydrophilic capping agents to improve their solubility and stability. Various nanocarriers, including nanoemulsions, dendrimers, micelles, liposomes, solid lipid nanoparticles, and nanoparticles of biodegradable polymers, can be employed for delivery of the active agents. For example, zein, sodium caseinate (NaCas), and pectin were exploited as polymeric matrices to formulate colloidal complex nanoparticles for the encapsulation of eugenol
[45]. Small (~140 nm under optimized conditions), uniform, and stable particles were obtained. The process was affected by synthetic conditions and, preferably, pH 6.6 corresponding to the isoelectric point of zein should be maintained.
Analogously, pH-responsive biopolymeric nanocapsules containing essential oils of
Thymus vulgaris,
Rosmarinus officinalis, and
Syzygium aromaticum were formed with the use of chitosan, alginate-chitosan, guar gum-chitosan, xanthan gum-chitosan, and pectin-chitosan
[46]. The average encapsulation efficiency in nanovessels of 100–500 nm was 60% while the loading efficiency was 70%. Essential oil release kinetics were determined in pH = 3, 5.6, and 7.4. The best results were obtained for nanocapsules built from a chitosan-guar gum structure. The chitosan-guar gum and chitosan-pectin nanocapsules released 30% of essential oils (similar release kinetics for thymol, eugenol, and α-pinene) at pH 3 and 80% at pH = 7.4 during 3 h. Growth rates of
S. aureus and
E. coli, as well as their minimal inhibition concentration values, were estimated. The MIC of
Thymus vulgaris and
Syzygium aromaticum essential oils ranged from 0.025 to 0.5%.
Release kinetics of thymol, cinnamaldehyde, and eugenol encapsulated in liquid lipid nanoparticles (LLN) or solid lipid nanoparticles (SLN) were studied as a function of the lipid structure and carrier particle concentration in pullulan-based films
[47]. The EO significantly reduced the crystallization temperature of SLN, and their release rate from SLN films was twice as high as that of LLN films. The release rate also depended on the type and amount of active compound (thymol > eugenol > cinnamaldehyde).
Eugenol and linalool were also encapsulated in polylactic acid
[48]. Their antimicrobial activity against
E. coli,
Salmonella enterica,
S. aureus, and
Listeria monocytogenes was studied, and their minimum inhibitory concentration and minimum bactericidal concentration were evaluated. MBC values of these strains were, respectively, 0.39%, 3.13%, 0.78%, and 1.56% (for eugenol) and 0.39%, 12.50%, 0.39%, and 12.50% (for linalool). Inhibition zones of relevant size were detected for EUG against
E. coli (60 mm) and linalool against
Salmonella (32 mm). The capsules have prolonged efficiency, continuing to release the active species for up to 40 days. Such bio-based antimicrobial systems can be potentially applied as active food packaging.
The slow release of natural antimicrobials from nano-vessels can also improve the performance of bacteria-repellent polymer coatings. A hemocompatible interpenetrating network designed to repel bacteria was prepared with the use of poly(lauryl acrylate) nanocapsules containing eugenol
[49]. The vesicles were entrapped within the network and EUG was slowly released. A significant reduction in surface-bound
Klebsiella pneumoniae and methicillin-resistant
S. aureus (MRSA) was achieved with this system when the hybrid material was coated on a catheter and an endotracheal tube.
This entry is adapted from the peer-reviewed paper 10.3390/antibiotics12111570