Studies have reported a concerning trend of heterogeneous mass loss in the HKH region for the past few decades. A thorough study of HKH glacier extent changes
[3] reveals a slow and consistent retreat trend of Hindu Kush glaciers, especially in China, India, Nepal, and Pakistan, and a rapid retreat trend of more than the global average in Himalayan glaciers. However, over the Karakoram range, glaciers exhibit a more diverse response with comparatively less change and more stable conditions. A multi-temporal analysis and comparison study from 1991 to 2014 shows that the glaciers of the Ladakh range, Jammu and Kashmir, India, have shrunk by −12.8% (−45.3 km
2)
[4]. From 1968 to 2019, the Swetvarn and Thelu glaciers in the Rakvarn glacial valley of the Bhagirathi basin, Garhwal Himalaya, India, retreated by about 16.60% and 24.50%, respectively
[5]. The largest glacier in the Sikkim Himalaya, Zemu, shrank by 30.67 ± 2.87% at a rate of 0.35% per year, and its snout retreated by ~797 ± 19.7 m at a rate of 9.1 ma
−1 during the last 87 years between 1931 and 2018. However, the retreat rate has increased to ~20 ma
−1 lately, from 2014 to 2018. A total of 147 glaciers from the Kashmir Himalaya show a notable loss of 28.82% (29.32 ± 12.09 km
2) of covered area during the last 38 years. These glaciers covered an area of around 101 ± 16.79 km
2 in 1980, which shrank to 72.41 ± 4.7 km
2 in 2018. This recession is synchronous with the temperature increase during the period from 1980 to 2018
[6]. According to reports
[7][8], 65 glaciers situated in the five mountain ranges of the North-Western Himalaya (the Karakoram range, the Zanaskar range, the Ladakh range, the Shamaswari range, and the Greater Himalaya) have shrunk to 1073 ± 24.6 km
2 area in 2014 from 1106 ± 33.6 km
2 in 1990, and the glacier volume has reduced to 211 ± 4.85 km
3 from 219 ± 6.66 km
3 during this period. Based on the glacier inventory prepared by the International Centre for Integrated Mountain Development (ICIMOD), Kathmandu, Nepal, 885 glaciers of the Bhutan Himalaya show around 23.3 ± 0.9% glacial cover fall-off between ~1980 and 2010, with a minimum fall-off of around 6.7 ± 0.1% (2000–2010) and a maximum of around 11.6 ± 1.2% (~1980–1990)
[9]. Between 2000 and 2012, almost 12,243 glaciers with a 19,727 ± 1054 km
2 area in the Upper Indus Basin of the Jammu and Kashmir Himalaya thinned by around −0.35 ± 0.33 ma
−1. The highest and marginal thinning of −1.69 ± 0.60 ma
−1 and −0.11 ± 0.32 ma
−1 were observed in Pir Panjal and Karakoram glaciers, respectively. This thinning of glaciers is also influenced by topographic parameters
[6].
In the western Himalayas, the total glacier cover of 77 glaciers in the Dras basin has dropped by 5.31 ± 0.33 km
2 with a mean snout retreat of 155 ± 9.58 m (30 m to 430 m range) from 2000 to 2020
[7]. In the Chandra Basin, the glacier area dropped off to 608 ± 10.3 km
2 in 2016 from 639.4 ± 5.8 km
2 in 1971 at a rate of 0.7 ± 0.3 km
2 per year, resulting in a total loss of 4.9 ± 1.9%, equivalent to 31.3 ± 11.8 km
2 [10]. Reports show a 2.5% loss in glacier cover from 377.6 km
2 to 368.2 km
2 from 1980 to 2010
[11]. The average glacier snout retreated by 465.5 ± 169.1 m at an average rate of 15.5 ± 5.6 m per year during this period. Such extensive mass loss of Himalayan glaciers will have significant direct consequences for freshwater availability, irrigation, and hydropower generation, and can also increase the risk of glacial lake outburst floods (GLOFs) throughout Asia.
The large-scale glacial retreat observed worldwide since the latter half of the 1900s is widely attributed to global warming and climate change. Climatic elements such as monsoon circulation, wind patterns, temperature, and precipitation exhibit distinct characteristics between the western and eastern Himalayas, with the Tibetan Plateau also influencing their glacier retreat patterns. According to the projections of the Intergovernmental Panel on Climate Change (IPCC), the Asian land mass is expected to experience an average annual mean warming of approximately 3 °K by the 2050s and around 5 °K by the 2080s. However, it is anticipated that the Tibetan Plateau will undergo even more significant temperature increases
[12]. The tropospheric warming trends between the western and eastern Himalayas and the Indo-Gangetic (IG) plains exhibit inequality in the magnitude of tropospheric warming trends in these regions
[13]. Ice core studies from the Himalaya-Tibet region have identified the role of the South Asian monsoon and the mid-latitude westerlies in influencing the Himalayan glacier mass balance over millennial timescales and the intensified heating caused by dust and anthropogenic pollution, including black carbon, in the Indo-Gangetic (IG) plains, Himalayas, and Tibetan Plateau, affecting the temperature gradient and monsoon circulation
[14][15][16]. Understanding the intricacies of climate change and their interaction with glacial dynamics is essential for devising effective policies and strategies to safeguard the Himalayan region and its dependent communities.
2. Atmospheric Temperature Trend (MSU/AMSU)
In order to estimate multidecadal changes in global atmospheric temperature, measurements from two different measuring systems, balloon-borne radiosondes and satellite-borne microwave-sounding equipment, have been largely used
[17][18][19][20][21]. The Microwave Sounding Unit (MSU) and Advanced Microwave Sounding Unit (AMSU) were flown on a series of polar-orbiting weather satellites launched by NOAA in 1978 and 1998, respectively. The microwave measurements are constructed by merging measurements from both and are referred to as the MSU dataset. These satellite-based instruments provide measurements processed by different organizations and agencies to produce valuable data products that contribute to our understanding of climate dynamics, weather forecasting, and the detection of long-term temperature trends. The satellite microwave sounding observations of the lower troposphere and middle troposphere since 1979 show a rise in global temperature of 0.156 °K/decade and 0.091 °K/decade, respectively
[22][23].
By incorporating the observed prevalence of vertically extended atmospheric brown clouds over the Indian Ocean and Asia into general circulation model (GCM) simulations
[24], it is suggested that these clouds contribute significantly to regional lower atmospheric warming trends. The study proposed that the observed retreat of Himalayan glaciers could be attributed to the combined warming trend of 0.25 °K/decade associated with atmospheric brown clouds and greenhouse gas emissions. The findings indicate that the observed trend of warming in the mid-troposphere across west-central Asia in recent years has significant implications for the amplified variability of western disturbances and an elevated likelihood of intense winter precipitation events in the western Himalaya region
[25]. However, the precise implications of this climate warming signal on the hydrological cycle of the HKH region are yet to be understood.
The long-term temperature trends in two cities, Delhi and Kolkata, in the Indo-Gangetic plains based on radiosonde data from 1973 to 2008 reveal that both cities experienced warming trends in the lower troposphere until 400 hPa, with the maximum increase occurring at 700 hPa
[26]. A notable shift from cooling to warming trends occurred between 1958–1985 and 1973–2008. The study suggests that increased emissions from fossil fuels, urbanization, and aerosol distribution, including greenhouse gases and particulate matter, may have influenced these temperature trends, but further comprehensive observations are needed to establish their specific roles. Using radiosonde data, researchers in India have managed to examine the variability of annual and seasonal tropospheric temperature changes from 1971 to 2015
[27]. It was reported that the all-India mean annual temperature has experienced significant increasing trends at surface, 700 hPa, and 500 hPa levels, driven primarily by pronounced winter warming. Surface air temperatures in India have shown significant increases across all seasons. North and South India exhibit varying temperature trends, with surface and 700 hPa levels showing significant increases, influenced by differences in aerosol concentrations between the two regions. The layer average temperature was growing in the lower troposphere (surface–850 hPa) and falling in the lower stratosphere (100–50 hPa) in a trend study of 10 years of Aqua-AIRS sounding atmospheric temperature data over a few major Indian cities
[28]. The decreasing trend in the lower stratosphere was found to be prominent. A literature review of the lower and middle tropospheric temperature trend studies in the HKH region using MSU datasets is tabulated below (
Table 1).
Table 1. Microwave Sounding Unit/Advanced Microwave Sounding Unit (MSU/AMSU) temperature trend over some of the regions in the Hindu Kush-Himalayan (HKH) region. TMT: temperature middle troposphere; TLT: temperature lower troposphere
[29].
3. Climate Change Indicators: Glaciers
There has been a general trend of glacial retreat since the Little Ice Age, similar to many mountainous regions worldwide, but from the 1990s on, glacial retreat was more accelerated than during any other period in the 20th century
[33]. On a regional scale, the glacier loss in the HKH region is influenced by its distinct climate variations, as the eastern part of the region experiences monsoonal-dominated precipitation during the summer, while the western part is influenced by mid-latitude westerlies bringing precipitation in the winter
[34]. Individual glacier observations reveal that annual retreat rates differ from one basin to another, with some glaciers even advancing. The central Himalayan glaciers (from Uttarakhand to Bhutan) are receding at different rates than the western Himalayan glaciers
[35]. The study also shows that the deglaciation rate in the Bhaga basin, western Himalayas, has drastically increased to 0.25% per year in the past two decades (2000–2020), compared to 0.12% per year in the previous decades (1971–2000). The Machoi glacier, distributed between the Greater Himalayan Mountain Range of Kashmir and Zanskar, experienced a shrinkage of 1.88 km
2 area, equivalent to 29%, with a frontal retreat of 500 m at a rate of 10.6 ma
−1 from 1972 to 2019
[36]. In the Trans-Himalayan Kang Yatze Massif, Ladakh, Northwest India, around 121 small glaciers have decreased in the area from 96.4 km
2 in 1969 to 82.6 km
2 in 2010
[37]. The loss in the glaciated area is 14% at a rate of 0.3% per year. Recent studies have reported shrinkage of glacier areas and surface elevation from 1975 to 2015 in the Kanchenjunga region of the Himalaya, which is attributed to increased air temperature
[38]. The average change rates observed in the glacier area were −0.18 ± 0.07% a
−1 and in the surface elevation were −0.32 ± 0.02 ma
−1.
However, in the past two decades, the Karakoram mountains have been reported to be growing and showing stable or positive glacier budgets, known as the Karakoram anomaly
[39][40][41]. A mass balance study of 19 glaciers covering 60% of the Astore basin, north-western Himalaya, using satellite images and DEM between 1999 and 2016 shows no net mass loss in that period, exhibiting a pattern similar to the Karakoram
[42]. The inhomogeneous glacier response pattern across the Himalayas is linked to the interaction between increasing temperatures and the local topography
[43]. The mean snow cover peak is observed in February, and the trough is in August and September from 2000 to 2017 across the entire HKH region
[44]. Conducting a comprehensive examination of the long-term trend in upper air temperature within the high-altitude regions and evaluating its interplay with climatic dynamics would facilitate the comprehension of the fluctuations observed in the spatial distribution of snow and glacier coverage across the HKH region (
Table 2).
Table 2. Characteristics of the major glaciers and snow-covered regions in the Hindu Kush-Himalayan (HKH) region
[29].
4. Importance of MSU/AMSU Datasets
The spatial distribution of meteorological stations providing long-term air temperature data is limited, especially in Himalayan and Tibetan regions, resulting in a lack of comprehensive coverage beyond their immediate vicinity
[68]. This spatial data scarcity poses a significant obstacle when examining temperature variations across these heterogeneous regions. The ability to monitor the temperature of the planet and its atmosphere from orbit is made possible by remote sensing sensors. The data collected by Microwave Sounding Unit (MSU) and Advanced Microwave Sounding Unit (AMSU) sensors provide the longest continuous record of tropospheric temperature derived from satellite sensors. A dataset spanning over 45 years has been generated using measurements of brightness temperatures captured by the MSU and AMSU, which allows for long-term climate studies and trend analysis. This extensive dataset provides information on atmospheric temperatures across four distinct layers, including the lower, middle, and upper tropospheric and lower stratospheric regions.
MSU data sets have emerged as a potentially valuable tool for monitoring global temperatures. However, it monitors a physically different quantity than surface temperature. There is a fairly strong correlation between the temperature anomalies from the MSU and global surface datasets
[69]. Recent studies have found a consistent and robust cooling trend of approximately 1–3 °K in the stratosphere as well as a consistent and robust warming trend of about 0.6–0.8 °K in the troposphere over the period of four decades from 1979 to 2018
[70]. The results remain consistent when comparing satellite-based average temperature measurements (MSU datasets) with vertically resolved radiosonde records. As most of the Himalayan high terrain plateau is situated above 4 km, the recorded tropospheric temperatures in this area primarily correspond to the mid-tropospheric temperature measured by the MSU.
The temperatures in mountainous areas are rising at a faster pace compared to the global average
[71]. The glacial mass balance trends in the HKH region, being extremely sensitive to temperature changes, call for a holistic assessment of the long-term tropospheric temperature changes over the region and the factors inducing them.
The present study
[29] investigates the tropospheric temperature trends (lower and middle) over the Himalayan (western and eastern), Tibetan Plateau, Indo-Gangetic (IG) plains, and the major dust source regions in northern mid-latitudes that influence the Himalayan and IG plains
[72] (
Figure 1).
Figure 1. The sub-division of the study region into high-altitude regions (Tibet, western and eastern Himalayas) and plains (western and eastern Indo-Gangetic plains (IG plains)). The major dust source regions of Asia-Africa affecting the IG plains and Himalayas are marked as Afghanistan-Pakistan, Iran-Pakistan-Thar desert, Middle East, Arabian region, and Sahara Desert
[29].
5. Discussion
The mid-troposphere temperature (TMT, 3–7 km altitude) trend can be practically considered the lower troposphere temperature (TLT, 0–3 km altitude) trend over the high-altitude HKH region, where the average elevation is ~5 km. The TMT trend over the dust source, dust sink, and surrounding snow-covered and glacial regions (Himalayas and Tibetan Plateau) shows a statistically significant mean warming trend. The observed warming trends align with the overall warming of the tropical troposphere observed globally during the same period [70][73]. The HKH region is reported to show a warming of almost 1.8 °K by the end of the century[74].
The high-altitude glacial regions exhibit enhanced warming compared to other low-lying regions (Figure 2). The Tibetan region shows the highest warming trend, followed by the eastern Himalayas and the western Himalayas, respectively.
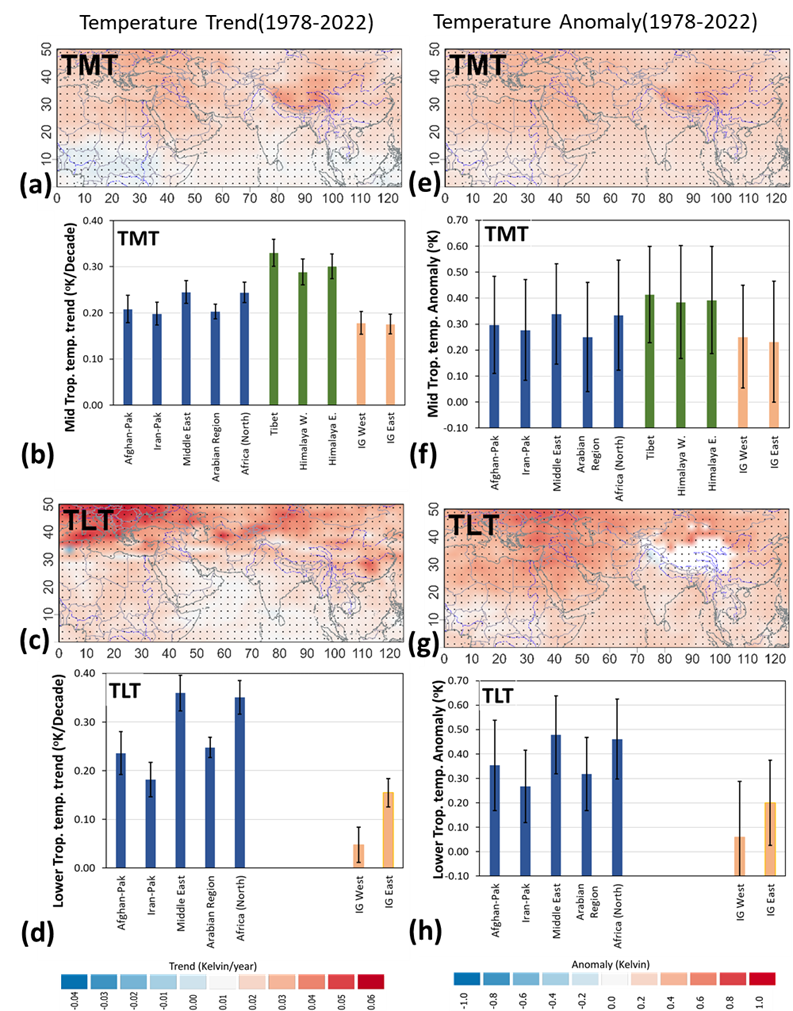
Figure 2. The overall mid-troposphere and lower troposphere temperature (TMT/TLT) trend map (°K/year) (a, and c), anomaly map (°K) (e, and g), and its corresponding trend/decade (°K/decade) and anomaly (in °K) chart (b, f, and d, h) over high-altitude Tibet, Himalayas (western and eastern), adjacent Indo-Gangetic (IG) plains (western and eastern), and dust source regions (as marked in Figure 1). The dots on the trend maps indicate that the linear trend over the region (pixel or grid) is statistically significant.
The monthly breakup of warming trends exhibits that the high-altitude glacial regions clearly show enhanced warming during winter and pre-monsoon months (November to May) and a decline during the other half of the year with a relatively neutral or slightly positive trend.
In general, an inequality of warming trend can be observed between the western and eastern Himalayas, where the western Himalayas show a relatively higher warming trend in pre-monsoon months (peak in May, 0.019 ± 0.005 °K/year) compared to other months, while the eastern Himalayas show much higher warming trends in winter months (peak in December through February, average of 0.041 ± 0.012 °K/year) compared to pre-monsoon months.
Previous studies had reported the unequal distribution of TMT warming with enhanced warming of the western sides of the Himalayas and IG plains compared to the eastern sides in the pre-monsoon months [13][31]. Significant dust storms originating from arid and desert areas in Africa, Arabia, and western India, including the Thar Desert, occur during the pre-monsoon season (April–June) in the Indian Gangetic (IG) plains [72][75]. These dust storms, along with locally produced anthropogenic pollutants, affect the entire IG plains during the pre-monsoon. The frequency and impact of these dust storms are higher in the western region and relatively lower in the eastern part of the IG plains. These dust-laden air masses form a layer of aerosols along the foothills of the Indian Himalayas, contributing to the “elevated heat pump” (EHP) mechanism [16][75]. This mechanism enhances convection, causing the vertical advection of dust aerosols to higher altitudes, increasing the seasonal heating during pre-monsoon (MAM) months, and resulting in a decreasing gradient of aerosol optical depth (AOD) towards the east [76][77]. Though the seasonal anomalous heating of the western Himalayas and IG west during MAM months was distinctly identified by the study, the disappearance of the decreasing TMT gradient from west to east and the strong jump in TMT trends in the eastern Himalayas and IG plains are conspicuous.
The annual TMT trends derived in the present study[29] for the IG west and IG east exhibit similar degrees of warming trends, in contrast to the earlier works on the TMT trends derived for the IG plains. The annual TMT trends of the Himalayas also show a similar pattern, with not much contrast between the western and eastern Himalayas. Previous studies had identified unequal annual TMT warming trends between the western (western Himalayas, IG west) and eastern (eastern Himalayas, IG east) sides of dust sink and snow-glacier cover regions from MSU-derived TMT trends for the time period 1979–2008, with the western Himalayas and IG west showing a much higher annual TMT trend than the eastern Himalayas and IG east [13]. The present study[29] clearly reveals that the warming of the middle troposphere in the eastern dust sink and snow-glacier cover regions has alarmingly increased over the past decade.
The changes in western disturbances over the western Himalayas suggest that significant warming of the Tibetan region in recent decades will result in stronger temperature differences between the middle and upper troposphere over the sub-tropics and mid-latitudes, causing the mean westerly winds to become more unstable, leading to greater variability in the occurrence of weather disturbances and an increased likelihood of heavy precipitation events over the western Himalayas[25]. The enhanced warming of the Tibetan region over the past decade, as observed in the present study[29], could explain the increased winter precipitation and the anomalous advancement of glaciers in the western Himalayas.
6. Conclusions
The highlights of the study[29] are:
- The study emphasized the acceleration of mid-troposphere warming trends in the HKH region compared to neighboring IG plains.
- A comparison of TMT trends between 1979–2008 and 1978–2022 revealed significant rises in warming over the last 14 years:
- Tibet: ~3.1 times
- Eastern Himalayas: ~1.7 times
- Western Himalayas: ~0.8 times
- IG west: Nearly zero rise
- IG east: ~0.4 times
- Dust source regions: ~1.3 times
- Overall, the findings suggest an increase in regional mid-troposphere temperature trends across the HKH region, IG plains, and western dust source regions over the 45-year period.
- Warming trends in TMT showed a distinct increase in high-altitude snow-glacier-covered regions (Tibet, western Himalayas, and eastern Himalayas) compared to adjacent plains (IG west, IG east).
- Seasonal variations were observed in TMT warming trends, with a strong increase during pre-monsoon months in the western Himalayas and IG plains, attributed to heightened dust storm activity.
- The divergence in snow and glacier melting rates between the western and eastern Himalayas, as well as Tibet, was attributed to distinct regional tropospheric warming patterns.
- The research emphasized that higher TMT warming anomalies and trends during November–April (NDJFMA months) led to increased glacier and snow cover melting during the primary snow accumulation season from November to February (NDJF months) in the Indian subcontinent.