1. Neuroinflammation and Microglia Dysregulated Genes in AD and the Effects of Phytochemicals
The role of glia activation and other immune cell dysfunction is well described in AD
[1]. Single-cell RNA sequencing revealed AD-associated brain transcriptome alterations in specific types of microglia
[2]. Peripheral blood single-cell RNA sequencing also uncovered enhanced immune cell signatures and reduced B cell-related molecular biomarkers in AD
[3][4]. Additionally, in the search for the identification of the underlying mechanisms of immune cell dysfunction, large-scale whole genome DNA methylation analyses of post-mortem brains and blood samples identified that DNA methylation alterations associated with AD are involved in the dysregulation of the immune system
[5]. Moreover, while there is evidence for accelerated epigenetic aging in AD
[6] and by using an “epigenetic clock,” one can reliably estimate cellular/tissue aging
[7], more recent studies revealed that the loss of epigenetic information is among one of the key components of events contributing to cellular aging
[8][9]. In other studies focusing on important microglia innate immune receptor genes, an increase in the DNA methylation of TREM2 in the superior temporal gyrus was linked to AD pathogenesis
[10]. Nevertheless, another study reported that the increased DNA methylation of TREM2 associated with a >3-fold increased expression in the hippocampus was due to the enrichment in 5-hydroxymethycytosine (5-hmC) at the TREM2 gene body
[11] where, in general, 5-hmC increases the associated gene expression. There is also a report on the increased TREM2 expression in the blood cells of patients with AD associated with the DNA hypomethylation of several CpGs in its intron 1
[12]. However, there is also evidence that the increased TREM2 expression in the hippocampus might be a secondary protective reaction. In fact, the overexpression of TREM2 using adeno-associated virus vector gene delivery increased the number of Iba-1/Arg-1-positive microglia, suppressed neuroinflammation and microglial activation, and improved cognition in high fat-fed mice exhibiting glucose intolerance as well as learning and memory problems
[13].
Several studies indicate that phytochemicals could attenuate neuroinflammation and improve cognitive performance involving TREM2 in mice models of AD. For instance, in APP/PSEN1 transgenic AD model mice, it has been shown that cognitive dysfunction and hippocampal neuroinflammatory responses are decreased by Bilberry anthocyanins and microglia phagocytosis of beta-amyloid protein plaques is improved through upregulating TREM2/TYROBP/CD33 signaling pathway [22]. In a more recent study, Cyanidin-3-O-glucoside (C3G), a phytochemical found in fruits and vegetables, could also reduce neuroinflammation and reactive oxygen species (ROS), and enhance microglial Aβ42 phagocytosis through upregulation of TREM2 in mice model of AD [23].
Other key elements of the immune system in AD are complements C1q, C3, and C4 [24,25], along with TGFB2 [26] which regulates synapse pruning [27]. As shown in Figure 1, Aβ protein activates astroglia NFκb and releases the complement C3 which acts on neuronal complement C3a receptors (C3aR) and disrupts dendritic morphology and network functions in AD patients [28]. Other studies reported that complement C3 and C3aR1 are increased by aging, which results in inflammation and increases the permeability of the vascular structure (mediated by Ca++), impacting the blood-brain barrier (BBB) integrity associated with lymphocyte infiltration, and greater microglial activity [29]. Furthermore, as the expression of complement C3 and C3aR1 in human AD brain are correlated with cognitive decline, the inactivation of C3aR attenuates tau pathology, neuroinflammation, and neurodegeneration in mice models of AD [30].
Notably, aging is also associated with DNA hypomethylation and reactivation of transposon elements such as LINE1 and SINE which induce inflammation. Transposons are viral DNA that has been incorporated into the genome of different species and compromise almost 50% of the human genome. Epigenetic mechanisms, including DNA methylation, are responsible for suppressing their activity throughout the life of any animal. It has been shown that SIRT6 is one of the key genes in transposons inactivation via inducing DNA methylation, and its upregulation suppresses transposons reactivation leading to longer life in Drosophila melanogaster [37]. In humans, its overactive allele is associated with longer life, i.e., becoming a centenarian [38]. Fucoidan, a phytochemical of Atlantic brown algae, increases SIRT6 expression by nearly 14-fold [39], increases lifespan and is neuroprotective in D. melanogaster [40,41], and improves LPS-induced cognitive impairment in mice [42], thus a promising anti-aging phytochemical.
Besides SIRT6, SIRT1 expression is also downregulated by brain internal factors/toxins such as Aβ accelerating neuronal cell senescence, which can be attenuated by exogenous SIRT1 expression as well as aspirin (salicylic acid, a plant hormone) that upregulates SIRT1 expression [43]. Phytic acid was also shown to diminish the dysfunction of these players in cell culture experiments and the aged mice brain [44]. In addition, it has been shown that the active compounds of black chokeberry (Aronia melanocapa L.) decrease the expression of several inflammatory factors, including ITGB2/C3R, in neuronal cells [45]. As ITGB2 is upregulated in blood mononuclear cells of AD patients, 1α,25(OH)2-vitamin D3 (1,25D3) could promote Aβ phagocytosis by macrophages of AD patients and decrease inflammation in vitro [46].
Regarding TGFβ2, while its overexpression is reported in the neurons of patients with AD [26], in vitro studies uncovered that its expression is induced by toxic Aβs both in glial and neuronal cells. Interestingly, the increased TGFβ2 protein in the brain binds to the extracellular domain of Aβ precursor protein and activates a neuronal cell death pathway in AD, and the degree of TGFβ2-induced cell death is greater in cells that express a familial AD-related mutation in APP versus those that express the wild-type APP [26,47]. There is evidence that the expression of TGFβ2 can be downregulated by certain phytochemicals (e.g., withaferin A and active fractions of golden-flowered tea) in other diseases, suggesting their potential use in AD [48-50]. In neuronal tissue, curcumin exhibits anti-inflammatory effects and suppresses reactive gliosis in animal models of spinal cord injury through decreasing TGFβ1 and TGFβ2, along with proinflammatory cytokines such as TNF-α, IL-1β, and NF-κb [51]. High Mobility Group Box 1 (HMGB1), another gene linked to microglia function, is also involved in AD pathogenesis [52]. As HMGB1 is a known marker of neuroinflammation, and the serum level of HMGB1 is higher in AD [53], it may disrupt the BBB functions [54]. Tau oligomer also induces HMGB1 release promoting cellular senescence [55]. Quercetin and naringin are known to suppress HMGB1 and ROS or proinflammatory cytokines in other diseases [55,56]. Galangin could suppress the HMGB1/TLR4 bond mitigating astrocytic activation and neuroinflammation in rat brains [58]. In other studies, it was shown that HMGB1 expression is regulated by HDAC4&5 and miR-129 in brain cells [61,62]. As miR-129 is known to regulate neuronal migration in mice brains [63], choline (abundant in spinach) upregulates miR-129-5p expression in neural progenitor cells both in vitro and in vivo [64]. Other phytochemicals (e.g., gallic acid and sulforaphane) could influence HDAC4 or HDAC5, thus opening new windows for more studies in the treatment of AD and other neurodegenerative diseases. YAP and TAZ genes (members of the superfamily of ATP-binding cassette transporters) whose activities decline during aging are other key elements of cellular senescence or aging. As YAP/TAZ, through the suppression of cGAS/STING, preserve nuclear envelope integrity involving innate immunity and prevent aging, inhibition of STING (stimulator of interferon genes or TMEM173) prevents tissue aging and senescence-associated inflammation [65]. PQBP1-cGAS-STING pathway is also involved in Tau-induced microglia activation leading to brain inflammation [66]. It has been shown that supplements containing NAD+ (and its precursors abundant in mushroom, avocado, and cucumber) could reduce the expression of proinflammatory cytokines and mitigate microglia and astrocytes activation through decreasing cGAS-STING activity which finally attenuates neuroinflammation and cell senescence in a mouse model of AD [67]. The cGAS-STING signaling could be inhibited by Astin C (a cyclopeptide extracted from the plant of Aster tataricus) as well, which decreases the innate inflammatory responses triggered by cytosolic DNAs both in vitro and in mice [68]. Figure 1 and Table 1 show key affected genes and a summary of findings related to the effects of different phytochemicals with potential application in neurodegenerative diseases. However, there are other phytochemicals that exhibit neuroprotective effects via other mechanisms. For example, quercetin exhibits neuroprotective effects through the downregulation of α-synuclein protein aggregation in PD [14] and inhibits the fibril formation of Aβ proteins in AD [15]. Similarly, naringenin could alleviate the neurotoxic effects of Aβ in vitro associated with the downregulation of the expression of APP and BACE, reducing amyloidogenesis, and decreasing the phosphorylated tau level [16]. Several other lines of evidence also support the beneficial effects of naringenin in AD and PD [17], as well as the neuroprotective effects of Bacopa monnieri extract, with potential therapeutic applications in AD [84].
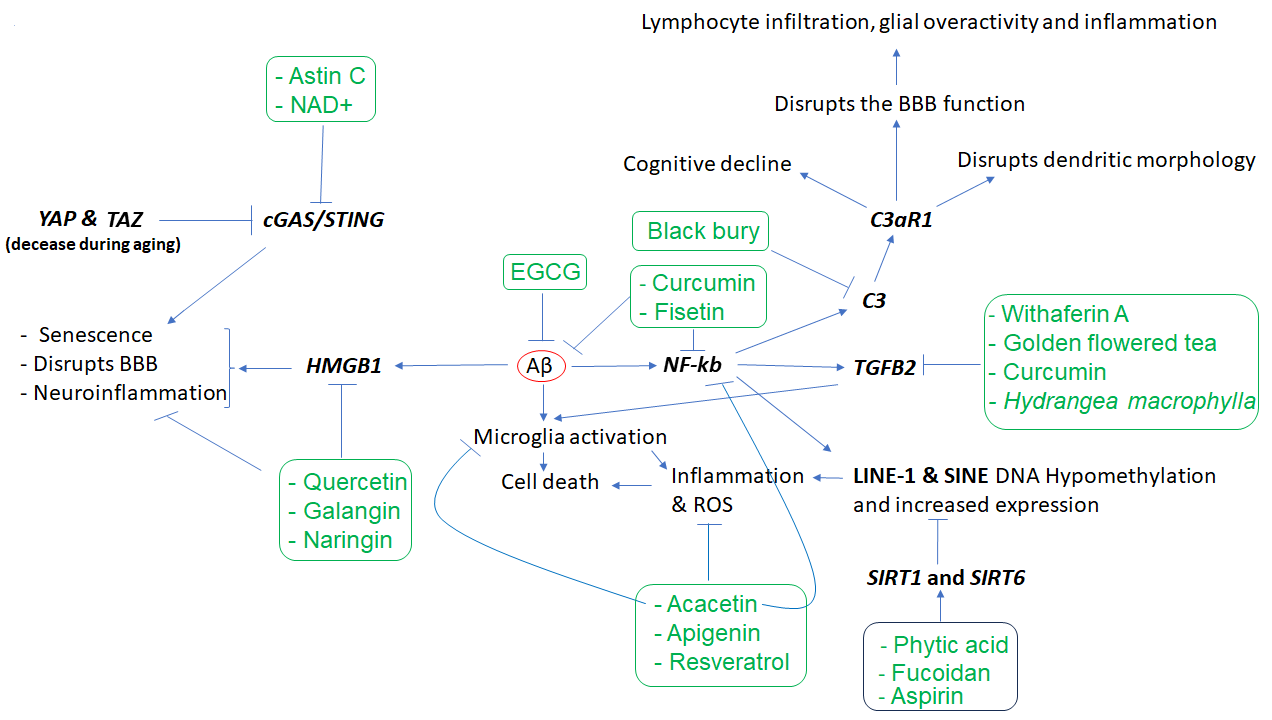
Figure 1. The cascade of events resulting from amyloid-beta (Aβ) accumulation in the brain and the phytochemicals that may mitigate the effects of these events. Aβ appears to play a central role in inducing inflammation, which is mediated by the overactivity of HMGB1, NKB, C3, C3AR, and TGFB2, as well as LINE-1 and SINE transposons, among others. However, several phytochemicals (inside rectangles) have the potential to target the affected genes, reduce inflammation, and mitigate the other cellular dysfunctions that contribute to neuronal death. In the figure, arrows indicate stimulation, while the T-shape marks denote inhibition. Genes are presented in bold.
Table 1. Phytochemicals that modulate neuroinflammation and brain aging-related genes in neurodegenerative diseases.
2. Brain Neuronal Stem Cells (NSCs) in Aging and the Effects of Phytochemicals
Aging is associated with a decrease in the total NSC population in the hippocampus (from >20,000 in each square millimeter at age 3 months to almost 7000 at the age of 12 months in mice). In addition, the quiescent NSCs (qNSCs), which upon activation, enter a primed quiescent state from the dormant state and generate neurons and glia, enter a deeper quiescence state due to aging
[36]. Thus, the recovery potential of the aging brain is compromised.
It has been shown that NSC proliferation is increased by homolocarpum seed oil (a rich source of α-linolenic acid and β-sitosterol), thus indicating it is a potential candidate to counteract brain aging
[37]. Likewise, several other phytochemicals, such as daucosterol, a component of walnut meat, also boost NSC proliferation mediated by increases in IGF expression and AKT phosphorylation in cell culture experiments
[38].
Alyssum homolocarpum seed extract also increases NSC proliferation in mice brains
[39]. Furthermore, Kuwanon V isolated from the root of a mulberry tree (
Morus bombycis) was shown to increase neurogenesis (from rat NSCs) and cell survival, mediated by the reduction in extracellular signal-regulated kinase 1/2 phosphorylation, increase in p21 expression, Notch/Hairy downregulation, and the upregulation of the microRNAs miR-9, miR-29a, and miR-181a
[40]. Silibinin, a polyphenolic flavonoid from
Silybum marianum, also increases NSC proliferation in mice through BDNF/TrkB signaling transduction
[41]. Similarly, a resveratrol pretreatment could increase NSC survival and proliferation and decrease the apoptosis associated with the upregulation of Nrf2, HO-1, and NQO1 protein expression, as shown following an oxygen–glucose deprivation/reoxygenation challenge in vitro
[42]. Additionally, curcumin was shown to inhibit the bisphenol A-mediated reduction in NSC proliferation and neuronal differentiation by activation of Wnt/β-catenin signaling in the mouse hippocampus
[43]. However, in cell culture experiments, curcumin was shown to inhibit NSC differentiation and increase cell survival mediated by decreases in Atg7 and p62 expression, the markers of autophagy
[44]. Neuroprotective effects of capsaicin and resveratrol against Glu-induced toxicity were shown in the cerebral cortical neurons of mouse fetuses as well
[45].
In more recent studies, it has been shown that while key genes encoding basic transcription factors for stemness are active during embryogenesis, they are silenced in later life. However, concurrent increases in the expression of SOX2, OCT4, and KLF4 have been associated with recovering the lost epigenetic information in aged cells and the rejuvenation of several tissues, including neuronal cells in mice eyes [95]. Therefore, the induction of the expression of these genes was shown to be a promising therapeutic approach for the treatment of age-related diseases via recovering epigenetic memory [17,95]. Several phytochemicals are known to potentiate the expression of these genes. For example, di-(2-ethylhexyl) phthalate (DEHP), a C. vulgure phytochemical, increases SOX2 expression in hippocampal NSC of mice in vitro associated with increased cell growth rate [96]. Furthermore, sulforaphane (a phytochemical compound of broccoli), Withaferin A (a steroidal lactone from a medicinal plant), and Betulinic acid (a phytochemical from several tree bark extracts) could increase the expression of KLF4 in vitro [97-99]. Although many phytochemicals reduce the expression of OCT4, short-term low-dose ethanol treatment (1 week, 1-5 mM, equivalent to the blood concentration of ~0.0048-0.024%) increases OCT4 expression several folds in vitro [100]. Therefore, an appropriate combination of these phytochemicals with low-dose ethanol may help to increase the expression of these key transcription factors posited to be useful in tissue rejuvenation or regeneration.
3. Telomere Attrition and Aging and the Effects of Phytochemicals
The shortening of telomere length, which usually occurs during cell replication, is another determinant of aging. It is known that psychological stress, chronic infections, mitochondria dysfunction, and ROS reduce telomere length (
Figure 2). For instance, chronic inflammation due to CMV, HIV, herpes, and hepatitis B and C infections shortens telomeres’ length
[46]. One of the important functions of lengthier telomeres is the expression of long non-coding RNAs regulating the expression of NF-kb, MYC, VEGF, and DNMTs involved in inflammation
[47]. Telomere length also affects the expression of many other genes by looping and other mechanisms
[47].
Figure 2. Different factors are involved in balancing telomere length. Telomerase activity helps maintain telomere length, while various factors can decrease telomere length. On the other hand, several phytochemicals and the Mediterranean diet have been shown to increase telomerase expression or activity, thereby potentially increasing or maintaining telomere length.
While telomerase enzymes protect against telomere shortening, and cells with more telomerases have longer telomeres, the short-term use of cooked
brassica leafy vegetables could increase telomerase activity in CD8+ lymphocytes in humans
[48]. Moreover, there is evidence that the chemical compounds of
Astragalus membranaceus activate telomerase, inhibit senescence, and have neuroprotective effects
[49].
Eucalyptus camaldulensis bark extract increases telomerase expression, exhibiting anti-aging, anti-apoptosis, and anti-senescence effects as well
[50]. In an in silico study, acacetin-7-O-β-D-glucoside, a compound of
Thunbergia erecta, was also shown to increase telomerase activity in addition to inhibiting acetylcholinesterase (AChE), suggestive of its possible use for the treatment of AD
[51]. However, it is important to note that, similar to stem cells, telomerase activity is higher in almost 90% of human cancers
[52]. Hence, there have been concerns that using phytochemicals or drugs to increase telomerase activity or expression might have double-edged sword effects. Meanwhile, recent studies found that the non-conical functions of the telomerase TERT subunit include the reduction in mitochondrial oxidative stress, DNA damage, and apoptosis, as well as neuronal degradation induced by toxic proteins such as α-synuclein, Aβ, and pathological tau, along with the activation of neurotrophic factors and autophagy, all contributing against age-related neurodegenerative diseases
[53]. In this line, recent animal and in vitro studies support that a Jing Si herbal drink increases autophagic clearance in neurons and maintains stem cell homeostasis while suppressing cancer cell growth and migration
[54].
4. Gut Microbiome, the Dysfunction of Brain Microglia and Astrocytes in Brain Aging, and Phytochemical Effects
Similar to the brain, the enteric peripheral nervous system also contains glial cells, which are reactive to the gut microbiota composition, infection, and inflammation
[55]. While dysfunction of glial cells has been shown in neurodegenerative diseases (described above), an altered gut microbiome has been repeatedly reported in AD and PD
[56][57][58][59]. Furthermore, it has been shown that fecal transplantation from old mice to young mice impacts their performance in spatial learning and memory tests. This is associated with the altered expression of hippocampal proteins involved in neurotransmission and synaptic plasticity along with the aging-like phenotypes of the recipient mice microglia in the hippocampus fimbria
[60].
Regarding the mechanisms that are involved in the functional status of different tissues/cells as the result of microbiome alteration, it has been shown that the gut microbiota community affects blood inflammatory cytokines. For example, one study from Finland reported a reduced abundance of
Prevotellaceae (almost 75%) in PD
[61] and another study from Taiwan reported a less abundance of
Prevotella (a genera of
Prevotellaceae) but more abundance of
Verrucomicrobia,
Mucispirillum,
Porphyromonas,
Lactobacillus, and
Parabacteroides in the feces of patients with PD
[58]. These microbial alterations were correlated with the plasma levels of IFN-γ and TNF-α in the patients
[58]. In particular, the fecal abundances of
Bacteroides and
Verrucomicrobia were highly correlated with TNF-α and IFN-γ levels, respectively
[58]. Interestingly, another study reported that a larger number of microbial 16S rRNAs are present in the serum of patients with Parkinson’s disease compared to control subjects
[62]. This indicates that specific gut bacteria may cross the intestinal wall (likely due to a leaky gut) and induce inflammatory reactions leading to increased serum levels of inflammatory cytokines.
It is important to note that, as different communities live in diverse environmental milieus with different nutritional habits and genetics, it is conceivable that various communities exhibit different levels of vulnerability to the microbial compositions associated with specific diseases, yet there might be some similarities, as shown in
Table 2. For example, regarding PD, an increased abundance of
Lactobacillus was reported in the fecal samples of patients from Japan, Russia, and Taiwan but not in Finland and the USA. However, a decreased abundance of
Prevotellaceae has been reported in Finland, Russia, and Taiwan. Regarding AD, a study of Chinese patients reported increased abundances of
Bifidobacterium,
Sphingomonas,
Lactobacillus, and
Blautia and decreased abundances of
Odoribacter,
Anaerobacterium, and
Papillibacter versus the control subjects
[63]. However, in Turkey, higher abundances of
Bacteroides and
Prevotella were reported in AD patients
[64]. In Americans, the increased abundance of gut
Bacteroidetes (similar to Turkey),
Blautia (similar to the Chinese), and
Alistipes but decreased abundances of
Bifidobacterium (versus the Chinese) and
Firmicutes were attributed to AD pathogenesis
[57]. Likewise, another study in Americans found an increased proportion of
Bacteroides, along with
Alistipes,
Odoribacter, and
Barnesiella, and a decreased proportion of
Lachnoclostridium in elderly AD patients. Meanwhile, this bacterial profile represented a higher abundance of the taxa involved in proinflammatory states and a lower proportion of bacteria synthesizing butyrate, a short-chain fatty acid (largely produced from indigestible fibers) with well-known epigenetic effects
[56]. Several other lines of evidence also indicate that a dynamic interplay between food content and gut microbiota continuously affects epigenetic mechanisms. In fact, the interaction between food and the gut microbiome is essential for producing the necessary factors (e.g., folic acid, vitamin B12, choline, and SCFA) that contribute to epigenetic modifications
[65].
Table 2. Similarities and differences of microbiota alterations in Parkinson’s disease (PD) and Alzheimer’s disease (AD) in different countries.
Other studies have shown that microglia are key elements of the “gut–brain axis” in transmitting the impacts of gut microbiota alterations into the brain tissue. For instance, a recent study reports that “gut microbiota-driven brain Aβ amyloidosis in mice requires microglia” to become established
[71]. Conversely, treatment with specific bacterial species such as
Clostridium butyricum could prevent microglia activation and Aβ deposits and have been associated with a reduction in inflammatory cytokines and an improvement in cognitive functions in a mouse model of AD
[72].
Altogether, these lines of evidence indicate that abnormal gut microbiota may induce astroglia inflammation as well as inflammation-induced oxidative stress, which may alter the epigenetic landscapes of microglia or astrocytes, triggering brain pathologies. On the other hand, emerging evidence supports that probiotics, diet, and phytochemicals modulate gut microbial composition and could be useful in the prevention or treatment of AD and age-associated neurodegenerative diseases
[73][74][75][76]. For example, in a multicenter randomized, double-blind, placebo-controlled study, 12-week probiotic treatment in elder South Korean adults could improve mental flexibility and decrease their stress score, increase BDNF serum levels, and decrease the relative abundances of
Eubacterium,
Allisonella,
Clostridiales, and
Prevotellaceae in their feces
[77]. Furthermore, a recent systemic review concluded that while the Mediterranean diet was linked to a lower risk of AD and PD, the abundance of eight bacterial species associated with AD or PD was modulated by this type of diet
[78]. Moreover, an animal study revealed that the Qisheng Wan formula, which comprises seven herbal drugs, adjusted the diversity and composition of gut microbiota, decreased Aβ
1–42 deposition and NF-κb, TNF-α, and IL-6 expression, and improved cognitive functions in a rat model of AD
[79]. Poria cocos, a fungus in the family
Polyporaceae that improves cognitive functions, also improve gut dysbiosis along with reduction of Aβ formation and increase in Aβ clearance in a mouse model of AD
[80].
5. Metabolic Disease, Caloric Restriction, Physical Exercise, and Aging
As obesity is a well-known factor for AD pathogenesis
[81] and overeating results in insulin resistance and mTOR activation
[82], caloric restriction is a well-documented remedy to mitigate the aging process by reducing the concentration of glucose, lipids, and amino acids and increasing some metabolites such as NAD+ and AMP which modulate SIRT1, AMPK, mTOR, and IGF1 activities. It has also been associated with the improvement of mitochondrial physiology and hemostasis by influencing the transcription factors FOXO and PGC1a
[83]. Among commonly used drugs, metformin, an antidiabetic drug originally derived from the
Galega officinalis plant, could act on multiple pathways targeting aging and age-related diseases by activating AMPK and upregulating SIRT1, and inhibiting mTOR, ROS, and NF-kb signaling, among others
[84]. In addition to metformin, resveratrol, epicatechin, and the NAD+ precursor nicotinamide riboside (a vitamin B3 derivative) have been shown to improve mitochondria functions through similar mechanisms
[85][86][87]. Like metformin, physical exercise also increases AMPK, which upregulates IL15 in muscle, while both AMPK and IL15 activities are decreased by aging
[88]. Physical exercise further mitigates the aging process by attenuating age-related chronic and sterile inflammation and immunosenescence that affect mitochondrial functions, while it is intensified by mitochondria damage
[89]. In light of these findings, it is intriguing to note that, like physical exercise, certain phytochemicals, such as curcumin, could reverse the expression alterations of hundreds of genes affected in AD
[90].
6. Chromosome X Inactivation and Neurodegeneration
Since AD is more common in women
[91], there have been efforts to uncover the underlying mechanisms of this difference versus men. In this line, XIST, a long non-coding RNA that regulates the inactivation of one X chromosome in female cells, was found to be an important player involved in female AD pathogenesis. XIST expression is increased in the aged female brain and the entorhinal cortex of female patients with AD
[92]. Single-nuclei RNA sequencing revealed that XIST expression is elevated with age in the hypothalamic neurons of female mice and that it can be considered a powerful predictor of neuronal aging
[93]. It has also been shown that XIST induces Aβ accumulation and neuroinflammation in the AD mouse model
[94]. Although there is no study to confirm that phytochemicals may suppress XIST expression, one study reports that the expression of XIST correlates directly with the blood glucose levels in gestational diabetes mellitus
[95]. This suggests that caloric restriction using fiber-rich foods or the Mediterranean diet and efficient nutritional management of diabetes (which is associated with a higher risk of AD) may be promising approaches in suppressing XIST expression. However, more research is warranted to study the potential effects of specific phytochemicals on XIST expression in females.
7. Vascular System and Neurodegeneration
Dysfunction of the vascular system and endothelial cells is linked to neuronal degeneration as well. For example, it has been shown that in aged or irradiated mice, the production of TGFB1 is increased in the endothelial cells, causing neuronal stem/progenitor cell apoptosis, which can be inhibited by selective TGFB signaling inhibitors
[96]. Dyslipidemia can also lead to the senescence of endothelial cells, which, in addition to atherosclerosis, affects their integrity and permeability
[97], thus impacting the functionality of the BBB and influencing brain functions. Furthermore, complement C3, which is increased in the hippocampal astrocytes by aging, affects C3aR1 on the endothelial cell surface, causing inflammation and vessel permeability
[98]. As mentioned before, while withaferin A and active fractions of golden-flowered tea inhibit TGFB1 expression
[99][100], the active compounds of black chokeberry (
Aronia melanocapa L.) decrease the expression of inflammatory factors, including C3 receptors, in neuronal cells
[24].
This entry is adapted from the peer-reviewed paper 10.3390/nu15153456