High blood pressure arises from vascular dysfunction
[20][11]. Oxidative stress is a hallmark of hypertension, preeclampsia, and diabetes
[21,22,23][12][13][14]. Excessive reactive oxygen species (ROS) play an important role in vascular dysfunction and contribute to the pathogenesis of hypertension-related disorders
[24,25,26][15][16][17].
2. Cav1.2 in Vascular Smooth Muscle
2.1. Overview of Ca
v
1.2
L-type Ca
2+ (Ca
v) channels are heteromultimeric complexes comprising pore-forming α1c and auxiliary β, α2δ, and γ subunits
[27][18]. The α1c subunit possesses four repeat domains (I–IV) linked by intracellular loops and intracellular NH
2-/COOH-termini with each domain containing six transmembrane segments (S1–S6) (
Figure 1). The ion-conducting pore is formed by S5 and S6 and the loop between them, whereas the voltage sensor is located in the S4 segments
[28][19]. The intracellular COOH terminus, along with intracellular loops, plays important roles in Ca
2+-dependent inactivation, channel trafficking, phosphorylation and oxidation
[28,29,30][19][20][21]. Ca
v is activated by membrane depolarization and its activation allows Ca
2+ influx through the channel pore. The expression of the α1c subunit itself can form functional channels to conduct Ca
2+ ions, whereas the incorporation of auxiliary subunits promotes membrane α1c expression and alters biophysical properties of the channel
[28,31][19][22]. Ca
v channels are sensitive to blockades by dihydropyridines (i.e., nifedipine), phenylalkylamines (i.e., verapamil) and benzothiazepines (i.e., diltiazem)
[32][23]. There are four types of Ca
v channels (Ca
v1.1–1.4). Ca
v1.2 is predominantly expressed in the heart and in vascular smooth muscle
[33,34][24][25]. Ca
2+ influx through the channel is a primary trigger of vasoconstriction and also participates in transcriptional regulation. The auxiliary subunits β2, β3, and α2δ1 are expressed in vascular smooth muscle
[35,36,37,38][26][27][28][29]. β subunits, lacking membrane-spanning segments, are located intracellularly and interact with the α interaction domain (AID) on the I-II linker of the α1c subunit
[39][30]. The α2δ1 subunit is a single gene product bound together by disulfide bonds. Whereas the α2 subunit is extracellular, the δ subunit contains a single transmembrane segment
[40][31]. The diversity of Ca
v1.2 is also conferred by alternative splicing. Smooth muscle contains Ca
v1.2 exons 1, 8, 9 *, 31/32, and 33
[41,42][32][33].
Figure 1. Topology of Cav1.2. Cav1.2 is formed by the pore-forming transmembrane α1c subunit, the intracellular β-subunit, the extracellular α2 subunit linked to the transmembrane δ1 subunit in smooth muscle cells.
2.2. Regulation of Ca
v
1.2
2.2.1. Regulation by Auxiliary Subunits
The molecular composition of Ca
v1.2 in vascular smooth muscle cells includes the pore-forming α1c subunit and auxiliary β2/3 and α2/δ1 subunits. The β3 subunit appears to be the principal β isoform in vascular smooth muscle cells
[35][26]. Genetic deletion of the β3 subunit resulted in reduced α1c expression in mouse aorta and is associated with a reduction in Ca
2+ channel current and a slower inactivation rate
[35][26]. This genetic manipulation also attenuates angiotensin II-induced upregulation of Ca
v1.2 channels in mouse mesenteric arteries and the development of hypertension
[38][29].
2.2.2. Regulation by Protein Kinases
Protein phosphorylation, a covalent addition of the phosphate group to the side chain of serine, threonine, and tyrosine residues by protein kinases, is a common posttranslational modification to fine tune activities of receptors, ion channels and enzymes. Unsurprisingly, Ca
v1.2 is a target of protein kinases, and its activity is subject to the regulation by protein phosphorylation. Both α1c and β2 subunits are phosphorylated by protein kinases A (PKA), C (PKC), and G (PKG)
[29,46,47,48,49][20][34][35][36][37]. A variety of putative serine/threonine phosphorylation sites have been identified, yet their role in regulating Ca
v1.2 remains unsettled
[50][38]. In vascular smooth muscle cells, the regulation of Ca
v1.2 by PKA is controversial. PKA is found to either inhibit or enhance Ca
v1.2 activity
[51,52,53,54,55,56,57][39][40][41][42][43][44][45]. The stimulatory effect of PKA on Ca
v1.2 in vascular smooth muscle cells depends on anchoring adenyl cyclase 5 and PKA by A-kinase anchoring protein 150 (AKAP150) to the proximity of Ca
v1.2
[56,58][44][46]. Phosphorylation of Ser 1928 in the COOH-terminus of the Ca
v1.2 α1c subunit is required for PKA-stimulated channel activity in vascular smooth muscle cells
[56][44]. Activation of PKG exhibits inhibitory effects on vascular Ca
v1.2
[52,57,59,60][40][45][47][48]. PKG mediates nitric oxide-induced inhibition of Ca
v1.2
[61,62][49][50]. Activation of PKC by phorbol esters and by Gq-coupled receptors also potentiates vascular Ca
v1.2 activity
[63,64,65,66,67,68,69,70][51][52][53][54][55][56][57][58]. Basal Ca
v1.2 activity is evidently under the tonic control of PKC as PKC inhibition/PKCα depletion enhances Ca
v1.2 activity in vascular smooth muscle cells
[67][55]. Activation of PKG by nitric oxide (NO) suppresses Ca
v1.2 activity
[49][37]. Similar to PKA, PKC is anchored by AKAP150 to adjacent Ca
v1.2 to alter channel activity
[71][59]. Protein tyrosine kinase c-Src promotes tyrosine phosphorylation of the α1c subunit and enhances Ca
v1.2 activity, which is believed to participate in regulating smooth muscle contractility
[72][60]. c-Src via its SH
2 and SH
3 domains binds to the COOH-terminus of the α1c subunit
[70][58]. Y2122 in the COOH-terminus of the α1c subunit appears to be the major phosphorylation site of c-Src
[70,73][58][61]. In vascular smooth muscle cells, c-Src enhances Ca
v1.2 activity
[64,74,75,76][52][62][63][64]. Phosphoinositide 3-kinases (PI3Ks) are found to increase Ca
v1.2 activity in vascular smooth muscle cells
[77,78][65][66]. PI3Kγ potentiates Ca
v1.2 activity by facilitating plasma membrane translocation of α1c subunits and this effect is mediated by AKT/PKB-induced β2 subunit phosphorylation
[79,80,81,82][67][68][69][70]. Integrins also participate in the mechanotransduction process of pressure-induced myogenic tone
[83][71]. Integrin receptor activation is found to increase Ca
v1.2 activity via c-Src and PKA
[84,85,86][72][73][74].
2.2.3. Regulation by Small GTPases
The Ras superfamily of small GTPases (also known as small G-proteins) are cellular switches that regulate a variety of biological processes in living cells. They have been implicated in regulating Ca
2+ homeostasis and Ca
v1.2 is recognized as an important effector of the RGK subfamily (Rem, Rem2, Rad, and Gem/Kir)
[87,88][75][76]. Unlike vascular Ca
v1.2, direct phosphorylation of the cardiac Ca
v1.2 α1c subunit does not contribute to PKA-stimulated channel activity as mutating all PKA consensus sites in the α1c subunit fails to block the increase in Ca
v1.2 activity in response to β-adrenergic stimulation
[89,90][77][78]. Cardiac Ca
v1.2 is under tonic inhibition of Rad due to its association to both α1c and/or β subunits
[91,92][79][80]. β-adrenergic stimulation induces RAD phosphorylation, promotes the release of RAD from Ca
v1.2, and increase channel activity
[90][78].
2.3. Ca
v
1.2 and Myogenic Tone
Vascular smooth muscle cells in resistant arteries and arterioles possess intrinsic ability to contract in response to an increase in intraluminal pressure and to relax upon a decrease in intraluminal pressure
[97][81]. This phenomenon is defined as myogenic response and the steady-state of vascular smooth muscle cell contractility in these vessels is termed as myogenic tone. Myogenic tone sets the basal vascular tone and distribution of blood flow to and within tissues/organs. In principle, peripheral vascular resistance can be described by the Poiseuille equation: R = 8Lη/πr
4, where R is the resistance, L is length of the vessel, η is viscosity of blood, and r is the radius of the vessel. According to the Poiseuille’s law, peripheral vascular resistance is inversely proportional to the radius to the fourth power.
Vascular smooth muscle cells in resistance arteries/arterioles become depolarized in response to increased intraluminal pressure
[98,99,100][82][83][84]. Various mechanisms have been proposed to regulate myogenic tone. It is widely believed that pressure-induced membrane depolarization in vascular smooth muscle cells is instigated by mechanosensitive or stretch-activated cation channels including transient receptor potential (TRP) channels and epithelial Na
+ channels (ENaCs)
[101,102,103,104,105,106,107][85][86][87][88][89][90][91]. The membrane depolarization leads to increased [Ca
2+]
i and vasoconstriction
[99,108][83][92]. The increases in both [Ca
2+]
i and/or myogenic tone are blocked by the removal of extracellular Ca
2+ or by Ca
v1.2 blockers
[99,109,110,111,112,113,114,115][83][93][94][95][96][97][98][99]. These findings suggest that altered intraluminal pressure initiates myogenic tone via membrane depolarization and subsequent opening of Ca
v1.2. This notion is corroborated by findings from the genetic deletion of Ca
v1.2 α1c subunit in smooth muscle
[116][100]. Such a manipulation abolishes myogenic reactivity in murine tibialis arteries
[116][100].
3. Roles of Cav1.2 in the Pathogenesis of Hypertension-Related Disorders
3.1. Aberrant Vascular Tone in Hypertension-Related Disorders
Hypertension is associated with increased peripheral vascular resistance. As myogenic tone is the fundamental element of vascular tone, it is reasonable to speculate that myogenic tone is altered in hypertension-related disorders. In patients with hypertension, myogenic tone is increased in coronary arterioles
[110][94]. Increased myogenic tone is also noted in resistance arteries from the adipose tissue of paravertebral muscles of hypertensive patients
[130][101]. Commonly used rat models of experimental hypertension include the spontaneously hypertensive rat (SHR), stroke-prone spontaneously hypertensive rat (SHRSP), Milan hypertensive strain (MHS), vasopressin-deficient (Di/H) rats, two-kidney, one-clip (2K1C rats), and among others. Compared to the Wistar-Kyoto rat (WKY), myogenic tone of afferent arterioles and arcuate arteries from SHR kidneys is increased
[131,132][102][103]. Mesenteric arteries of SHR and MSH also exhibits higher myogenic tone than those of WKY and Milan normotensive strain (MNS), respectively
[133,134][104][105]. Elevated myogenic tone is observed in cerebral arteries/basilar arteries of SHR/SHRSP/Di/H compared to WKY and Di normotensive (Di/N) rats, respectively
[135,136,137,138][106][107][108][109].
Myogenic tone is increased in skeletal muscle resistance arteries of diabetic patients
[144][110]. Various animal models such as type I diabetic rodents induced by streptozotocin (STZ), obese/type II diabetic rodents induced by high-fat diet (HFD), type II diabetic HFD/STZ rodents, type II diabetic Goto-Kakizaki (GK) rats, and type II diabetic C57BL/KsJ-db/db mouse have been developed in diabetes research
[145,146][111][112]. Systemic vascular resistance (also known as total vascular resistance) and arterial blood pressure are increased in C57BL/KsJ-db/db mice, HFD rats, and GK rats
[147,148,149,150,151,152,153,154,155][113][114][115][116][117][118][119][120][121]. Mesenteric arteries of HFD mice, HFD/STZ mice, and diabetic (db/db) mice displayed higher myogenic tone compared to their counterparts
[58,144,155,156][46][110][121][122].
3.2. Dysfunction of Vascular Ca
v
1.2 in Hypertension-Related Disorders
As aforementioned, activation of Ca
v1.2 in vascular smooth muscle cells is essential for the development of myogenic tone
[99,100,109,110,113,114,116][83][84][93][94][97][98][100]. The enhanced myogenic tone in peripheral resistance arteries and arterioles in hypertension-related disorders suggests potential dysfunction of vascular Ca
v1.2. Indeed, this notion is substantiated by lines of evidence from functional studies. First, the increased myogenic tone in resistance arteries/arterioles of hypertensive and diabetic animals was normalized by the Ca
v1.2 blocker nifedipine
[58,132,181][46][103][123]. Whereas specific deletion of the mineralocorticoid receptor reduces KCl- and Ca
v1.2 agonist Bay K 8644-induced vasoconstriction of mesenteric arteries
[142[124][125],
182], Bay K 8644-induced contraction and increase in [Ca
2+]
i in 2K1C rat aorta is greater than in the control 2K rats
[183][126].
Animal models of hypertension-related disorders have provided mechanistic insights into the understanding of the Ca
v1.2 dysfunction in these disorders. Both aberrant expression of and dysregulation of Ca
v1.2 contribute to vascular Ca
v1.2 dysfunction. Various studies reveal increased protein expression of α1c
[37[28][29][127][128][129],
38,187,192,193], α2δ1
[37[28][128],
192], and β3
[37,38][28][29] subunits in mesenteric, femoral, and cerebral arteries of SHR and angiotensin II-infused C57BL/6 mice. Consistently, increased expression of Ca
v1.2 is associated with enhanced channel activity in vascular smooth muscle cells
[67,185,193,194,195,196,197][55][129][130][131][132][133][134]. The expression and activity of Ca
v1.2 are reduced in mesenteric arteries of aged mice lacking mineralocorticoid receptors in smooth muscle cells
[143][135]. Dexamethasone administration increases the expression of the cardiac Ca
v1.2 α1c subunit in rats
[198][136]. As expected, Ca
v1.2 activity in A7r5 cells is increased following chronic dexamethasone exposure
[199][137].
An increase in protein expression of the α1c subunits is also observed in cerebral arteries of STZ rats, which is associated with increased Ca
v1.2 activity and contraction to KCl
[203][138]. Ca
v1.2 also displays increased activity in vascular smooth muscle cells of cerebral and mesenteric arteries from STZ, GK, and HFD rats and db/db mice
[153,204,205,206][119][139][140][141]. Human arteries from diabetic patients have higher Ca
v1.2 activity due to increased phosphorylation of α1C at Ser1928 by PKA
[56][44].
Gal-1 is highly expressed in the placenta
[207][142]. Circulating Gal-1 level also increases during gestation
[208][143]. Given the critical role of Gal-1 in regulating Ca
v1.2 surface expression/activity discussed above, it is reasonable to speculate that the elevated Gal-1 in pregnancy may contribute to reduced uterine arterial myogenic tone by suppressing Ca
v1.2 surface expression/activity
[209,210][144][145].
4. Roles of Reactive Oxygen Species (ROS) in the Pathogenesis of Hypertensive Disorders
4.1. Overview of ROS
Reactive oxygen species (ROS) are oxygen-containing molecules naturally produced in cellular metabolism. ROS comprise free radicals such as superoxide anion (O
2•−) and hydroxyl radical (
•OH) and nonradical molecule hydrogen peroxide (H
2O
2). O
2•− is formed from the one-electron reduction of molecular oxygen (O
2). It is a precursor to a cascade of other ROS. Its dismutation, either occurring spontaneously or being catalyzed by superoxide dismutases (SODs), produces H
2O
2. Through the Fenton reaction, H
2O
2 can be reduced to
•OH. Moreover, the reaction between O
2•− and nitric oxide (NO) results in the formation of peroxynitrite (ONOO
−).
ROS are produced in different cellular compartments including mitochondria, endoplasmic reticulum (ER), lysosomes, peroxisomes, and plasma membrane
[23,213,214][14][146][147]. Nicotinamide adenine dinucleotide phosphate oxidases (NOXs) and mitochondria are the major sources of ROS
[215][148] (
Figure 32). NOXs are a family of transmembrane proteins that catalyze the transfer of electron donated by NADPH to molecular oxygen to form O
2•− [216][149]. NOXs comprise seven isoforms (NOX1-5 and dual oxidases (DUOX) 1 and 2). NOX1, NOX2, NOX4, and NOX5 are primary isoforms in vasculature
[217][150].
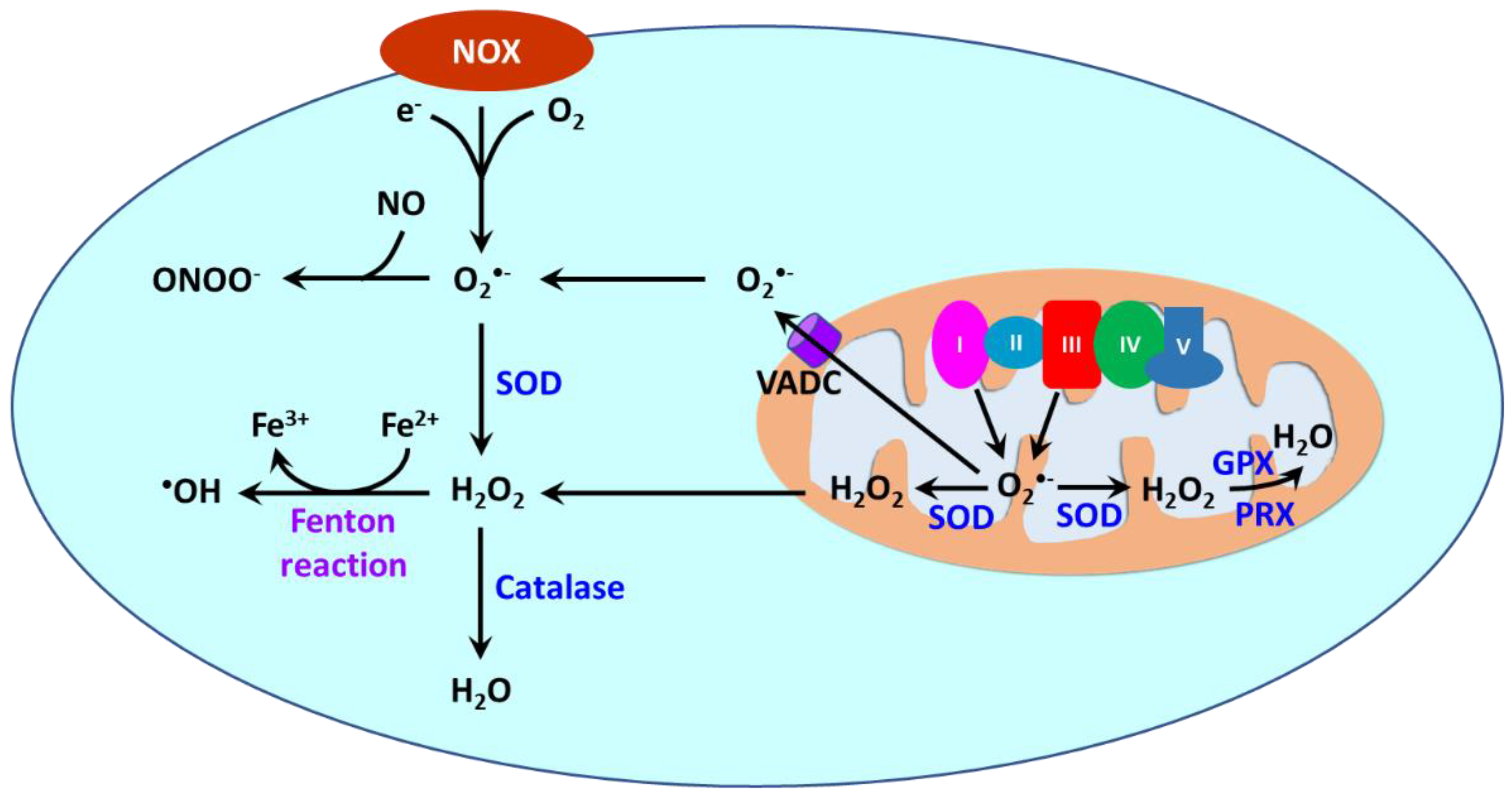
Figure 32. ROS generation by NOXs and mitochondria and detoxification. In vascular smooth muscle cells, ROS is primarily produced by NOXs and mitochondria. NOXs catalyze the production of O
2•− by transferring one electron to O
2 from NADPH. In mitochondria, O
2•− is also produced from leaked electrons by Complexes I and III in the electronic transfer chain (ETC) during the electronic transfer. O
2•− is dismutated to H
2O
2 by superoxide dismutase (SOD). H
2O
2 is subsequently decomposed to H
2O by catalase, glutathione peroxidase (GPX) and peroxiredoxin (PRX). O
2•− can be released into the cytosol from mitochondria via the voltage-dependent anion channel (VADC) on the mitochondrial outer membrane, whereas H
2O
2 in mitochondria can be transported to the cytosol via diffusion or via aquaporins. O
2•− reacts with nitro oxide (NO) to produce peroxynitrite (ONOO
−), whereas H
2O
2 reacts with Fe
2+ via the Fenton reaction to yield
•OH.
4.2. Oxidative Stress as a Hallmark in Hypertension-Related Disorders
Hypertension, diabetes, and preeclampsia exhibit a state of oxidative stress
[21,22,23][12][13][14]. A variety of studies have demonstrated that levels of biomarkers of oxidative stress such as O
2•−/H
2O
2, malondialdehyde, 8-isoprostaglandin F2α(8-iso-PGF2α), oxidized low-density lipoprotein (ox-LDL), and protein carbonyl are increased, whereas levels of antioxidants GSH (or GSH/GSSG ratio), vitamin C/E, and levels/activities of SOD, catalase, and GRX are decreased in the circulation of hypertensive
[224,225,226[151][152][153][154][155][156][157][158],
227,228,229,230,231], diabetic
[232[159][160][161][162][163][164][165][166],
233,234,235,236,237,238,239], and preeclamptic
[240,241,242,243,244,245,246,247,248][167][168][169][170][171][172][173][174][175] patients.
4.2.1. NOX-Derived ROS in Hypertension-Related Disorders
Several polymorphisms in the gene encoding p22
phox in human are associated with hypertension by affecting enzymatic activity
[310][176]. In SHR/SHRSP, the expression/activity of vascular NOXs 1, 2 and 4 are increased
[270,272,311,312,313,314,315,316][177][178][179][180][181][182][183][184]. DOCA rats have higher vascular expression of NOX subunit p22phox and enzymatic activity
[270,317][177][185]. NOX activity in cultured vascular smooth cells and in cultured rat mesangial cells is also stimulated by aldosterone, leading to increased ROS generation
[318,319][186][187]. Chronic administration of aldosterone causes NOX2-depednet increase O
2•− production in mouse cerebral arteries
[277][188]. Dexamethasone upregulates Nox1 expression in rat aorta via activation of the glucocorticoid receptor
[320][189]. In a mouse model of hyperadrenergic hypertension created by targeted ablation of the chromogranin a (Chga) gene, renal expression of NOX1/2 is increased
[321][190]. Angiotensin II-infused rodents are associated with elevated expression of NOXs 1 and 2 as well as NOX subunits p22
phox, p47
phox, and Rac1 in vessels and increased NOX activity
[268,274,322,323,324,325,326,327][191][192][193][194][195][196][197][198]. Angiotensin II apparently contributes to the upregulation of NOXs as it stimulates gp91
phox (NOX2) and p22
phox expression in vascular smooth muscle cells from human resistance arteries
[328][199].
In diabetic patients, vascular protein abundance of NOX subunits p22
phox, p67
phox, and p47
phox and enzymatic activity of NOXs are increased
[279][200]. Moreover, O
2•− production is reduced by non-selective NOX inhibitor diphenylene iodonium in vessels from diabetic patients
[279][200]. The expression and activity of NOXs 1, 2 and 4 as well as p22
phox/p47
phox are increased in aorta and mesenteric arteries of STZ rodents
[287,308,329,330,331,332,333][201][202][203][204][205][206][207]. Vascular expression/activity of NOXs 1, 2, and 4 as well as p22
phox are also elevated in db/db mice
[280,334,335][208][209][210]. Chronic high glucose exposure stimulates p22
phox, p47
phox and p67
phox expression and NOX activity in cultured endothelial cells
[285,286,336,337,338][211][212][213][214][215].
4.2.2. Mitochondria-Derived ROS in Hypertension-Related Disorders
Mitochondria become dysfunctional in hypertension-related disorders. SIRT3, a histone deacetylase, is an important regulator of mitochondrial redox state. It interacts with SOD2 in mitochondria and subsequently promotes SOD2 deacetylation, leading to enhanced enzymatic activity
[355,356][216][217]. Essential hypertension in human is associated with increased mitochondrial oxidative stress in arterioles from mediastinal fat due to reduced SIRT3 and increased SOD2 acetylation
[357][218]. Similar to human essential hypertension, SHR also exhibits overproduction of mitochondrial ROS in vessels
[358][219]. Partial deletion of SOD2 in mitochondria (SOD2
+/−) increases renal oxidative stress and blood pressure
[359][220]. In angiotensin II-infused mice, the increased blood pressure is associated with increased SIRT3 S-glutathionylation and vascular SOD2 acetylation, reduced SOD2 activity and elevated vascular O
2•− production
[357,360][218][221]. Those alterations are diminished by SIRT3 overexpression
[357][218]. Cyclophilin D is a regulatory subunit of the mitochondrial permeability transition pore and participates in regulating mitochondrial function
[361][222]. Evidently, it is an important mediator of angiotensin II-induced hypertension as its depletion diminishes both mitochondrial O
2•− generation in aorta and hypertension
[362][223]. Mitochondrial O
2•− generation in DOCA rat mesenteric arteries is also increased
[255][224]. In cultured HUVECs, excess aldosterone suppresses SOD2 expression and increases mitochondrial ROS production
[363][225].
Elevation of mitochondrial O
2•− is detected in subcutaneous arterioles in type 2 diabetic patients
[366][226]. Likewise, mitochondrial H
2O
2 is increased in primary human saphenous vein endothelial cells from type 2 diabetic subjects
[367][227]. STZ mice/rats, HFD mice, ZDF rats, and GK rats display increased mitochondrial ROS in vascular smooth muscle cells and endothelial cells
[331,368,369,370,371,372][205][228][229][230][231][232]. Hyperglycemia is apparently a causative factor leading to heightened mitochondrial ROS in vascular cells. Exposure to high concentrations of glucose stimulates mitochondrial ROS production in a variety of endothelial cells, promoting mitochondrial damage by producing 8-hydroxydeoxyguanosine and nitrotyrosine
[373,374,375,376,377,378,379,380,381][233][234][235][236][237][238][239][240][241].
4.3. A Causative Role of ROS in in Animal Models of Hypertension-Related Disorders
Oxidative stress is believed to be linked to pathogenesis and progression of a myriad of human diseases including hypertension-related disorders
[21,23,24,397,398,399,400,401][12][14][15][242][243][244][245][246]. Numerous studies using animal models reveal a causative role of ROS in the pathogenesis of hypertension, diabetes, and preeclampsia.
Treatment with the SOD mimetic tempol reduces vascular ROS and lower blood pressure in animal models of essential and secondary hypertension
[253,257,269,317,402[185][247][248][249][250][251][252],
403,404], of diabetes
[405[253][254][255][256],
406,407,408], and of preeclampsia
[293,294,346,409][257][258][259][260]. SOD1 deletion promotes development of hypertension, whereas the delivery of liposome-encapsulated SOD diminishes Ang II-induced hypertension
[267,410][261][262].
NOX inhibitors apocynin and diphenyleneiodonium have been shown to decrease vascular ROS, improve vascular function, and lower blood pressure in animal models of hypertension-related disorders
[272,275,312,317,324,340,411,412,413,414,415][178][180][185][195][263][264][265][266][267][268][269]. Angiotensin II-induced vascular ROS and hypertension is enhanced in transgenic mice overexpressing NOX1 in smooth muscle cells and is suppressed in NOX1 deficient mice
[274,325,416][192][196][270].
5. Contribution of Dysfunctional Cav1.2 Conferred by ROS to Increased Vascular Tone in Hypertension-Related Disorders
5.1. ROS and Ca
v
1.2 Function/Expression
There is ample evidence that Ca
v1.2 is regulated by cellular redox state. The α1c subunit contains 48 cysteines with some of them being sensitive to redox modulation
[420][271]. Redox modulation of sulfhydryl groups of cysteine residues could alter the structure and function of proteins. Site-directed mutagenesis has identified several cysteine residues including C543, C1789, C1790, and C1810 that subject to redox regulation
[421,422][272][273]. However, electrophysiological studies reveal conflicting effects of ROS on Ca
v1.2. Studies from Amberg’s group demonstrate that bath application of H
2O
2 and ROS produced by xanthine oxidase/hypoxanthine stimulates Ca
v1.2 activity in rat cerebral arterial smooth muscle cells
[423,424][274][275]. Both mitochondria- and NOX-derived ROS could exert stimulatory effects on Ca
v1.2. Antimycin enhances Ca
v1.2 activity by promoting mitochondrial H
2O
2 generation.
The redox modulation of Ca
v1.2 has immense impacts on vascular function. H
2O
2 is reported to trigger an increase in [Ca
2+]
i and vasoconstriction in rat mesenteric arteries, rat coronary arteries, rat aorta, and canine cerebral arteries
[429,430,431,432,433,434,435][276][277][278][279][280][281][282]. H
2O
2-induced increase in [Ca
2+]
i and contractile response are inhibited by the removal of extracellular Ca
2+ and by Ca
v1.2 inhibitors nisoldipine, nifedipine, diltiazem, and verapamil, suggesting that H
2O
2 activates Ca
v1.2 in vascular smooth muscle cells
[429,430,431,434][276][277][278][281].
NO is also a reactive, gaseous signaling molecule. S-nitrosylation, the covalent attachment of NO to a cysteine thiol in a given protein, is also an important redox signaling
[445][283]. S-nitrosylation of Cys 1180 and/or Cys1280 in Ca
v1.2 reduces surface expression of the channel and channel activity
[446][284]. In addition, S-nitrosylation of Ca
v1.2 also reduces channel open probability
[447][285]. Excess ROS leads to low NO bioavailability in hypertension
[448][286].
Remarkably, ROS also regulate gene expression
[460,461][287][288]. There is a concurring decrease in both vascular ROS and Ca
v1.2 expression in aortas of aged mice lacking the mineralocorticoid receptor in smooth muscle cells
[142][124]. The protein expression of the α1C subunit is increased by angiotensin II in cultured rat mesenteric arteries
[462,463][289][290]. H
2O
2 mimics, while NOX inhibition by apocynin, diphenyleneiodonium and gp91ds-tat as well as catalase annuls angiotensin II-mediated upregulation of the α1C subunit
[463][290]. In a cardiac muscle cell line HL-1 cells, angiotensin II upregulates the α1C subunit at both mRNA and protein levels through the NOX-PKC pathway-mediated cAMP response element binding protein (CREB) phosphorylation
[464][291]. It is expected that this mechanism could also play a role in secondary hypertension as hypertension due to Cushing’s syndrome, hyperaldosteronism, and renovascular disease involves activation of the renin-angiotensin system
[231,256,465,466,467,468,469][158][292][293][294][295][296][297].
5.2. ROS and Myogenic Tone
Exogenous ROSs have been shown to alter myogenic tone. In pressurized mouse tail arterioles and rat gracilis skeletal muscle arterioles, H
2O
2 causes vasoconstriction
[471,472][298][299]. The exposure of rat cerebral arteries to the ROS-generating system xanthine oxidase plus hypoxanthine also increases myogenic tone
[423][274]. Likewise, O
2•− generated by paraquat enhances myogenic tone in mouse afferent arterioles
[473][300].
•OH generated by the Fenton reaction from H
2O
2 and the iron redox chelate Fe
3+/nitrilotriacetate (FeNTA) elevates myogenic tone in denuded rat ophthalmic arteries
[474][301].
Numerous studies demonstrate that PKC/c-Src activation contributes to myogenic tone development in small arteries/arterioles
[136,487,488,489,490,491][107][302][303][304][305][306]. The expression/activity of vascular PKC/c-Src is increased in SHR and STZ rats as well as in uterine arteries of high-altitude pregnant sheep
[179,435,492,493][282][307][308][309]. PKC/c-Src activation has a greater contribution to myogenic tone in SHR cerebral arteries, STZ rat gracilis skeletal muscle arterioles, and high-altitude sheep uterine arteries
[136,159,179][107][307][310].
6. Conclusions
Evidently, Ca
2+ influx through Ca
v1.2 is fundamental to vasoconstriction and myogenic tone of small arteries and arterioles. Antihypertensive drugs including Ca
v1.2 blockers have been successfully used to the management of hypertension-related disorders
[495,496,497,498][311][312][313][314]. Oxidative stress is a hallmark of hypertension-related disorders. However, it is still an open question whether oxidative stress is a cause or consequence of hypertension. The data highlights critical roles of vascular oxidative stress in the pathophysiology of hypertension-related disorders. Vascular Ca
v1.2 is targeted by excessive ROS directly and indirectly leading to exaggerated channel expression/activity. The dysfunction of Ca
v1.2 ultimately results in increased myogenic tone and elevated blood pressure. In preclinical studies, ROS-induced myogenic tone is diminished by Ca
v1.2 blocker nifedipine
[473][300].