Polymer |
Chemical Structure |
Contact Angle with Water |
Young’s Modulus |
Application |
Polydimethylsiloxane (PDMS) |
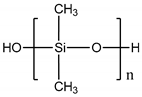 |
107° [78] | 107° [75] |
Variable from kPa to MPa |
Cardiovascular [79] Kidney [80] Liver [81] Lung [82] | Cardiovascular [76] Kidney [77] Liver [78] Lung [79] |
Gelatin |
extrusion |
kidney |
Poly (bisphenol-A- carbonate) (PC) |
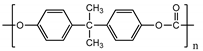 |
~85° [83] | ~85° [80] | [62,63] | [59][60] |
~1.2 GPa [ | 84 | ] | ~1.2 GPa [81] |
Tumor vasculature [85] Colon and breast cancer [86] | Tumor vasculature [82] Colon and breast cancer [83] |
Methacrylate gelatin (GelMa) |
extrusion |
Vessel, liver |
Poly (ethylene terephthalate) (PET) |
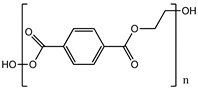 |
83° [87] | 83° [84] | [64,65] | [61][62 |
4.7 GPa [88 | ] |
] | 4.7 GPa | [ | 85] |
Gut [89] Kidney [90] Liver [91] | Gut [86] Kidney [87] Liver [88] |
Alginate |
extrusion |
Heart |
[66, |
Polylactic acid (PLA) | 67 | ] |
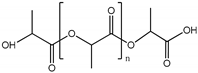 |
~75° [92] | ~75° [89][63] |
3.1 GPa [93] | 3.1 GPa [90][64] |
Endothelial barrier [ | 94 | ] | Endothelial barrier | [91] |
Cellulose |
extrusion |
tumor, liver |
Poly (ε-caprolactone) (PCL) |
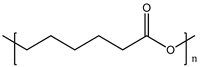 |
120° [95] | 120° [92] | [68,69] | [65] |
~400 MPa [96] | ~400 MPa [[66] |
Polyethyleneglycol (PEG) Poly ε caprolactone (PCL) |
inkjet, extrusion |
Colon tumor |
[59,65] | [56][62] |
2.2. Material Requirements for the Fabrication of Organ-on-Chips
Many biomaterials and fabrication methods have been recently developed to meet the OOCs’ functions. While designing the OOC microdevice, the organ or tissue characteristics and behavior must be considered to accurately simulate the in vivo motions, cell proliferation, or drug responses. Considering the organ characteristics aimed to be mimicked, different types of OOCs can be created.
However, although each type of OOCs may function differently, the main components remain the same. The OOC system is generally equipped with two compartments: first, the blood vessel compartment, which contains the endothelial cells; and secondly, the organ compartment, in which the cells of the investigated organ are introduced. These compartments are usually separated by a porous polymeric membrane able to provide cell communication between the two compartments of the device. Thus, the polymeric membranes play an essential role in the successful functioning of the OOC system, acting as interfaces with selective permeability for cell adhesion and cell separation.
The culture medium included in OOCs system may be artificially produced or naturally derived from living organs. The natural medium mainly contains biological fluids such as blood, plasma, etc., and organ fragments. The artificial environment is fabricated by involving nutrients that mimic the physiological conditions, such as salts, oxygen and CO
2 gasses, and blood substitutes. Thus, the cell-culture medium will provide a continuous supply of nutrients and the porous polymeric membrane will deliver the cells of interest that are cultured on the other side of the membrane. In addition to the nutrient-rich fluid perfusion and oxygen ventilation from the blood-vessel compartment, in the organ compartment, mechanical forces can be applied to the polymeric membrane to simulate the peristalsis respiratory motions from the in vivo organs.
Despite the significant progress in material science, only a few materials can recapitulate the physiological conditions of living organ testing. First and foremost, the materials involved in the construction of the OOCs microdevices should ensure the necessary biocompatibility to provide a biologically safe and nontoxic environment that allows cell migration without generating any inflammatory response or exacerbated immunogenicity. Polymeric materials are desirable because of their great structural similarity to the ECM.
Besides biocompatibility, an essential requirement for the polymeric membrane that separates the OOCs compartments is the similarity with the ECM in order to support the cell attachment and diffusion through its pores from one side of the interface to the other compartment. Proteins such as collagen, fibronectin, and vitronectin are ideal candidates as they are involved in biological processes and play an important role in the cell-adhesion mechanism. Moreover, it was shown that the surface roughness of the membrane could affect cell behavior. In the case of synthetic polymers such as PMMA layers, it was observed that the cell adhesion and spreading of vascular cells improved with higher surface roughness, but proliferation was not affected, and it was indicated that the cell adhesion is dependent on the protein adsorption
[70][67]. In the case of PDMS, surface roughness and surface energy play a key role in the cell-attachment process. It was observed that higher surface energy promotes the formation of stronger cell–ligand bonding, leading to improved cell growth and proliferation. However, the cell-membrane interaction drastically decreases above critical surface energy and critical roughness ratio. Thus, the study on the cellular behavior of HeLa and MDA MB 231 cells on a rough PDMS surface revealed that the optimum conditions for cell adhesion, growth, and proliferation are obtained at moderate surface energy and intermediate roughness ratios
[71]93 |
] |
Blood-brain barrier [ |
97 |
] | Blood-brain barrier | [ | 94 | ] |
Polytetrafluoroethylene (PTFE) |
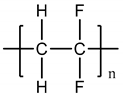 |
108° [98] | 108° [95] |
392 MPa [99] | 392 MPa [96] |
Liver [100] | Liver [97] |
As the surface properties such as surface roughness and wettability influence the first stages of cell adhesion and migration, the mechanical properties of the polymeric membrane are modulated by the material’s stiffness and influence the later stages of cell growth. Investigations such as tensile strength, compressive stress, and wettability are generally achieved to determine if the polymer properties are suitable for the application. Thus, the mechanical performances of the interface membrane from the OOCs should be chosen accordingly to the native organ characteristics and to mimic the occurring physiological stimuli present in the replicated environment. Moreover, the porosity of the membrane layer represents an essential feature as it provides cell communication between the chip compartments and allows nutrient transportation to the cells. Pore size and shape also determine the space available for the cells to migrate, and small pores mainly reduce the cell adhesion, but increase the barrier function of cells.
2.3. Polymers for Organ-on-Chips
Materials used to manufacture OOCs play a crucial role as they may directly interact with the biological fluids and cellular system, affecting the experiment results. Although several types of polymeric structures have been employed, only a few polymers exhibit the required specifications needed to fabricate the OOCs, and both synthetic polymers and biopolymers have been investigated.
In the last few years, synthetic and natural polymers have been used for OOC applications as their performances depend on the polymer structure, porosity, transparency, flexibility, stiffness, etc. Synthetic polymers such as polydimethylsiloxane (PDMS)
[101][98], polycarbonate (PC)
[102][99], polyethylenetereftalate (PET)
[103][100], aliphatic polyesters, polyurethanes
[104][101], etc., have been employed for the preparation of porous-compartment-separation membranes due to their easily adjusting porosity, surface roughness, and mechanical properties. Exhibiting improved biocompatibility, natural polymers such as collagen, gelatin, or polysaccharides have attracted significant attention lately due to their faithful replication of the native tissues with channel interconnections that allow the perfusion of oxygen and nutrients to simulate the natural behaviors of cell differentiation, spreading, and adhesion along the separating membrane
[105][102]. However, natural polymers show batch-to-batch composition variability and lower mechanical properties, thus affecting the reproducibility of the experiments. However, each employed biomaterial still exhibits weaknesses to achieve the optimal OOC performances.
Polydimethylsiloxane (PDMS) is an elastomeric polymer and one of the most employed synthetic materials for organ-chip production, as it exhibits optical transparency, biocompatibility, gas-permeation properties, flexibility, and allows permanent microscopic observation of 3D cell structures for real-time assessment of tumor behavior and response to therapy
[101,106,107][98][103][104]. Microfluidic systems made from PDMS offer a much closer physiological microenvironment to that of the living TME from a biomechanical point, exhibiting porosity and significantly lower stiffness. However, PDMS exhibits an important limitation in chemical screening due to its hydrophobicity, which leads to nonspecific absorption of small molecules such as drugs or pharmaceutical compounds
[108,109][105][106]. Nevertheless, the limitations of PDMS materials have been overcome through surface modification treatments with another polymer that enhances the hydrophilicity of the PDMS-based materials to facilitate cellular adhesion and migration in microfluidic compartments. One of the most commonly employed surface-modification approaches consists of oxygen-plasma treatment by converting some hydrophobic methyl groups into hydroxyl functionalities via oxidation. It was reported that the hydrophilicity of the oxidized PDMS after plasma treatment can increase by almost 30°
[110][107].
Moreover, the wettability behavior of PDMS materials can be improved by coating the polymer layers with other hydrophilic polymers. The proteins from ECM composition are generally used to achieve the natural moiety of PDMS to ensure cell attachment and proliferation. ECM proteins, such as collagen and fibronectin, create covalent bonding onto the PDMS surface and thus facilitate cell adhesion by modifying the surface roughness of the synthetic polymer
[111][108]. Although the hydrophilicity and biocompatibility are significantly increased this way, dissociation processes during protein coating are associated with this approach
[112][109]. Other biopolymers, such as gelatin
[113][110], fibronectin
[114][111], or hydrophilic synthetic polymers such as polydopamine
[115,116][112][113] and polyethylene glycol
[117][114] have been successfully employed for modifying the surface roughness of PDMS and managed to enhance the cellular adhesion for short-term cellular culture.
Polycarbonate (PC) structures are commonly used polymeric materials for the OOCs fabrication and as porous membranes for Transwell
® inserts. The PC structures are hydrophobic, exhibit high transparency, and are inert and stable under biological processes
[118][115]. Similar to PDMS, the surface of PC is commonly modified by gas plasma treatment or protein coating
[119][116]. However, PC structures exhibit higher rigidity than PDMS. They are not suitable for stretchable membranes or soft substrates.
Other thermoplastics are tested, such as polyolefins
[103][100], styrene-ethylene butylene styrene (SEBS)
[120][117], polyurethanes
[121][118], and copolymers
[122][119], but there is still a demand for novel materials in this domain.
Polyolefins such as cyclic olefin polymers (COP) and cyclic olefin copolymers (COC) are thermoplastic materials that have also been employed for the manufacturing of microfluidic devices, being less gas-permeable than PDMS. COP and COC are lipophobic materials and do not exhibit unspecific adsorption of small molecules, allowing their use in drug-development and diffusion experiments
[122][119]. Moreover, they exhibit optical transparency, good chemical resistance to polar solvents, thermal resistance, and reproductible mass production.
Polymethylmethacrylate (PMMA) is another frequently used material as a substrate
[123,124][120][121]. Kang and coworkers
[125][122] demonstrated that PMMA chemically attached to porous orbitally etched polyethylene terephthalate (PTFE) membranes is impermeable to small hydrophobic compounds and more consistent results concerning the anticancer vincristine drug cytotoxicity on human lung adenocarcinoma cells cultured in PDMS-based chip. Thus, PMMA shows promising features for tumor chip microdevices fabrication as the small molecules cannot infiltrate the material.
Aliphatic polyesters such as polylactic acid (PLA) and poly (ε-caprolactone) (PCL) have been used to mimic the in vitro blood–brain barrier, functioning as membranes in OOCs microdevices. Both polymers are biodegradable and hydrophobic. Although their surface can be chemically modified to enhance the wettability and cell proliferation, these polymers have been sparsely employed as they tend to degrade under biological processes releasing acidic degradation products that could affect the cellular medium
[123][120].
To fulfill the requirements of mechanical actuatable OOCs, the employed materials need to be optically transparent, flexible, easily moldable, and nonabsorptive to drugs and cell nutrients. Moreover, it is necessary to find sustainable alternative materials. At present, the manufacturing process of OOCs and experimental validation remain high-priced so that less-expensive and reusable materials should be employed.
Gelatin is an animal protein obtained by collagen hydrolysis, and is very much employed in drug-delivery and tissue-engineering applications
[126][123] due to its ability in drug transportation and cell-growth promotion
[127][124]. Gelatin exhibits biocompatibility and flexibility, and forms complexes with proteins, growth-factor nucleotides, and biopolymers, and can be shaped in colorless gels
[128][125]. Additionally, photo-crosslinked gelatin methacrylate (GelMa) is highly used in tissue engineering and shows interest in OOC formation
[129,130][126][127].
Collagen-based ECM gel (known as Matrigel) is a commercial product that contains ECM hydrogel made of tumor-derived basement membrane proteins employed for cell culture
[131][128]. Matrigel gives structural and signal-transduction functions. Tumor cells exhibit aggressive behavior in Matrigel medium, and it is frequently used to assess the malignancy of cancer cells and observe the mechanism of tumor growth
[132][129].
Bacterial cellulose paper has also attracted interest for tumor-chip applications as it has good biocompatibility, and it is a naturally derived polymer that is densely vascularized through nanofibers
[133,134][130][131]. Recent advances in bioprinting are multiplying the paths for creating complex perfused systems, and the discovery of new materials that accomplish the full requirements of native living cells is still under extensive development.