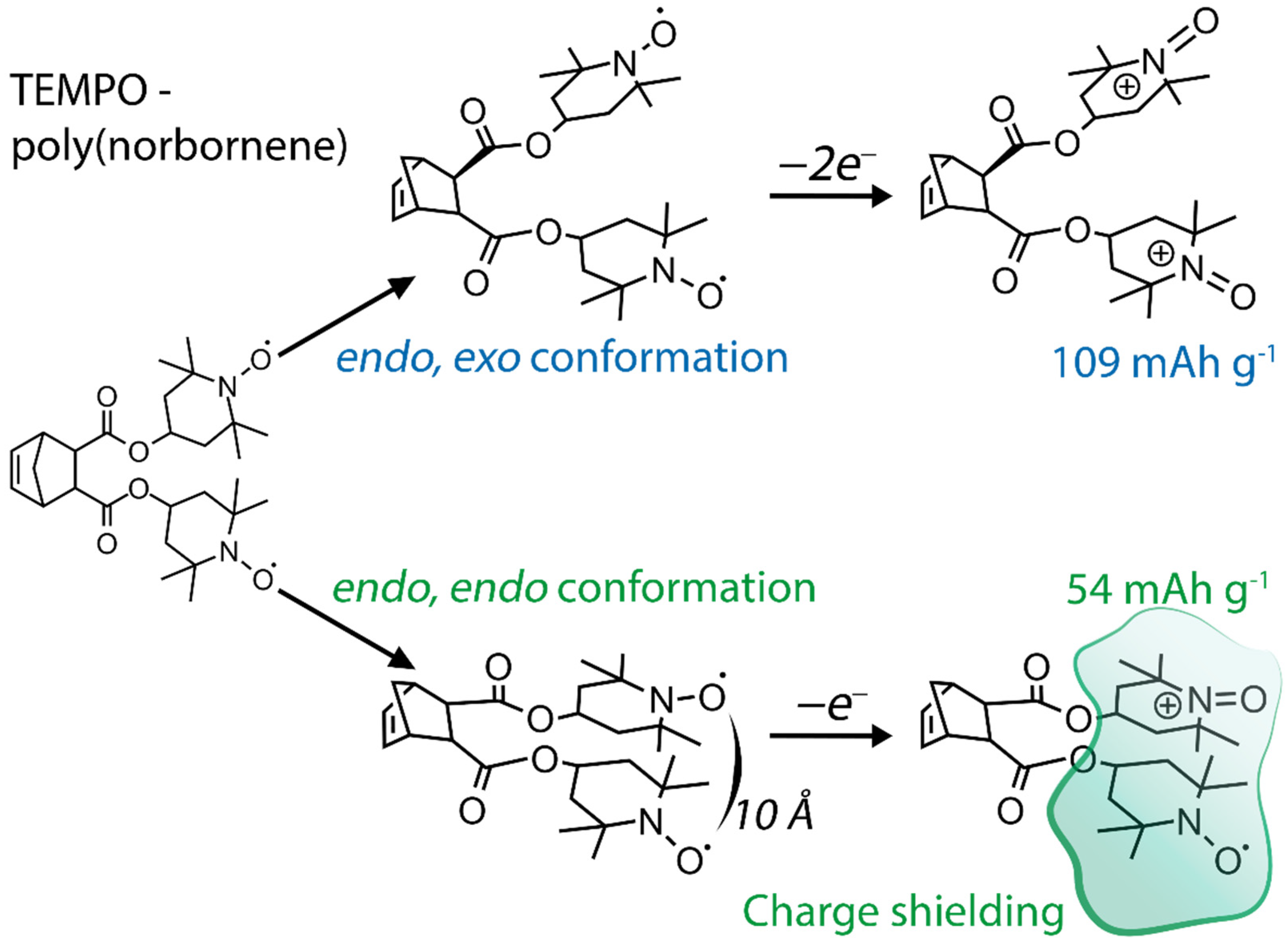
Figure 5. Schematic illustration of influence of neighboring TEMPO groups on molecule oxidation.
Another aspect worth considering is the intramolecular interactions for TEMPO substituents in long polymer chains, which require increased local concentration of charge carriers to make electron hopping more probable
[57]. One such option is annealing of a polymer with a TEMPO substituent, which results in formation of percolating networks, increasing the conductivity of the polymer to 10 S m
−1, compared to the 1 × 10
−9 S m
−1 of the initial disordered polymer with randomly oriented radical groups
[58].
High electron exchange rates notwithstanding, decent electrode materials performance also involves facile ionic transport. This is directly related to the following requirements to the electrode–electrolyte interactions: the polymer should swell, yet be insoluble, to prevent self-discharging of the electrode
[59], and the polymer should be either solvophilic or well-solvated to provide the mobility of the counterions.
The dissolution of the polymer may result in, e.g., 38% self-discharge in one week
[22], yet this might be easily prevented by crosslinking
[18] of the polymers.
Ensuring ionic conductivity requires tight control over both radical polymer and electrolyte properties. There are multiple factors at play here
[4][44][59]. The effect of the porosity of the material matters most in the case of thin-film electrodes, where the increase in thickness would hinder diffusion through the material, as bulk transport becomes the limiting factor
[60], which is observed already from the film thickness of 0.26 μm
[61]. The pores design may be improved via porous carbon architectures
[62], inclusion of porous polymers
[63], or development of three-dimensional electrode structures
[64].
To ensure electroneutrality, the process of electrochemical oxidation of NO• to N
+=O must be accompanied either by anion uptake or by cation expulsion, with the opposite process on reduction. This means that proper selection of material and electrolyte compositions should provide the conditions for high diffusion rates of charged species. Even for typical lithium-ion electrolyte, LiPF6 (in propylene carbonate, PC), the ratio between the number of TEMPO groups in PTMA and LiPF6 molecules strongly affects the performance of the material: changing the LiPF6-to-TEMPO ratio from 1.7 to 0.5 the self-exchange rate constant increases from 0.16 × 10
6 M
−1 s
−1 to 2.8 × 10
6 M
−1 s
−1 [65]. The decrease in the rate constant in highly concentrated electrolyte has to do with decreased mobility of ions—and predominantly with the nature of anions
[66][67][68]—in swollen polymers. It is worth noting, however, that cations also affect the performance of organic radical polymers, e.g., PTMA, and even participate in charge compensation, as shown by electrochemical quartz crystal microbalance
[69].
The replacement of the backbone polymer altogether may enhance ionic conductivity. For instance, (poly(2,2,6,6-tetramethylpiperidinyl-N-oxyl vinyl ether)) PTVE is hydrophilic, thus providing high affinity to aqueous electrolytes, and ensuring facile ion transport
[70]. Another example is (poly(2,2,6,6-tetramethylpiperidinyl-N-oxyl glycidyl ether)) PTGE, which has good swelling and ionic transport in standard 1.0 M LiPF6 in ethylene carbonate: diethyl carbonate (EC:DEC) electrolyte, providing up to 80 mAh g
−1 capacity at 10 C
[27].
RegAs a
rding the application side note, interactions of TEMPO
-containing compounds within the battery
, it should be considered that TEMPO may bear disruptive effects on other components by virtue of strong oxidizing capability of
itsTEMPO cation. For example, cellulose-based separators are likely to decompose in its presence
[71]. This presents an additional challenge when selecting optimal components.
Another way to improve the transport properties is to provide doping mechanisms via backbone polymer itself. This can be achieved by using conjugated polymers
[72][73], though the competing redox processes in radical and backbone components complicate the studies of the charge transport mechanism. Still, there is some understanding of such processes. For example, in TEMPO-modified polypyrrole (with acetylene black as conductive component)
[37] the charge transfer process is separated in four steps: (1) self-exchange between nitroxide radicals, (2) electron transfer from the radical to the conductive backbone, (3) electric conduction from polypyrrole to acetylene black, (4) electron transfer from acetylene black to the current collector (
Figure 6).
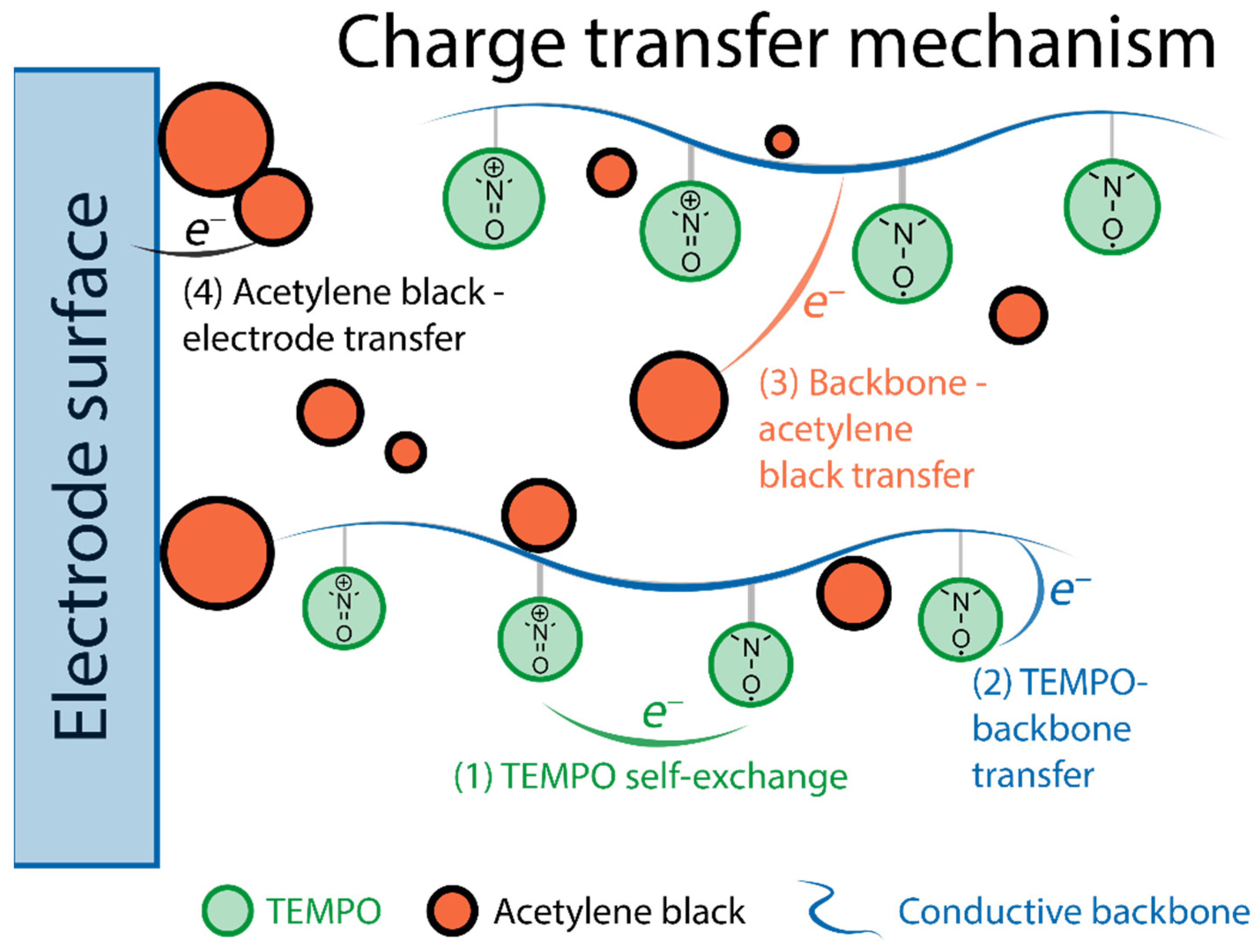
Figure 6. Schematic illustration of the charge transfer process in TEMPO-modified polypyrrole.
Polypyrrole is deemed to be an electric conduction path that assists repeatable oxidation/reduction through aggregated radical polymer bulks. The internal charge transfer between the TEMPO pendant groups and the backbone has also been observed in the case of TEMPO-modified 3,4-propylenedioxythiophene (ProDOT)
[74], though the precise effect of such interaction was not firmly established.
Adverse effects of TEMPO radicals
on the charge transfer wthin the electrode material have also been reported. In the case of TEMPO-modified poly(3-hexylthiophene) (P3HT)
[35], it turns out that intrinsic conductivity of P3HT—controlled both by intrachain and interchain hopping—is hindered by introduction of TEMPO. The impairing effect of TEMPO is due to introduced disorder in crystalline domains of the polymer, which restricts interchain hopping, thus terminating additional paths of charge transfer and decreasing conductivity. The effects of TEMPO content in P3HT on capacity were also investigated
[48], and, similarly, the capacity was the highest with the minimal content of TEMPO, while 100% TEMPO-loaded conjugated polymer provided both the lowest specific capacity and the highest charge transfer resistance. Another effect was observed for polythiophenes bearing pendant TEMPO groups
[39], where internal transfer of an electron from TEMPO to the polythiophene backbone caused rapid dedoping of the latter. This phenomenon led to a decrease in both capacity and conductivity and due to the thermodynamically favorable conditions: the 3.88 V oxidation potential of polythiophene is higher than that of nitroxide radical (3.60 V), thus facilitating backbone reduction. A reverse scenario, albeit with similar negative effects, was observed for poly(TEMPO−DTP) (TEMPO-modified (dithieno[3,2-b:2′,3′-d]pyrrole))
[75]: the low oxidation potential of poly(DTP) (3.15 V) caused electron transfer from the backbone to the radical upon charging, thus reducing the activity of the polymer and decreasing Coulombic efficiency. This means that the balance must be found in the thermodynamic preference of the reactions, so that the potentials of both charge carriers overlap in such systems.