An efficient approach to treating anthropogenic discharge (e.g., wastewater, effluents from agriculture and industry, stormwater runoff) is to create a constructed wetland (CW), which simulates natural marshland or swampland. These artificial wetlands employ physical, chemical, and/or biological methods, either consecutively or concurrently, to convert and extract different contaminants from the wastewater, and as such are highly intricate systems. Different system components of CWs, such as the porous bed, plants, and microorganisms, have contribution to the removal of phenols in wastewater treatment.
1. Introduction
The constructed wetland (CW) concept offers comprehensive removal of organics, nutrients, and solids with relatively low construction costs, and lower operating and maintenance costs than those associated with mechanical treatment technologies, especially for small- to intermediate-sized communities where suitable land is available. In addition, CW systems provide additional benefits, such as pleasing esthetics and wildlife habitats. However, purification efficiencies may vary depending on temperature, season, and geographical region.
A common CW is the subsurface flow CW—a depression lined with a porous bed (such as gravel) layer that underlies the effluent layer and supports a range of plants. The porous bed enables the separation of suspended solids from the effluent, in addition to the sedimentation stage which usually occurs at the entrance to the facility. Moreover, the extensive root surface of the plants allows the extraction of nutrients such as phosphate and nitrogen, as well as heavy metals and some of the less easily removed organic components
[1][2][3].
Most of the organic carbon components in CWs are removed by microorganisms, which usually exist in either planktonic (i.e., suspended) or sessile (i.e., as biofilms) form. Biofilms develop on the roots of plants or on the porous bed, forming specialized microniches and diverse communities; their activity differs greatly from that of conspecific planktonic cells, which are swept along with the water flow
[4][5][6]. These systems and their myriad components and effects on the environment have been described extensively by others
[7][2][3][8]. The interactions between the bacteria and the main system components—porous bed, plants, and effluent water—have been studied for some contaminants
[8][9][10][11]; however, knowledge about such interactions remains insufficient with respect to phenols. The main system components of a subsurface flow CW and an experimental method to monitor the partial contribution of each component are illustrated in
Figure 1.
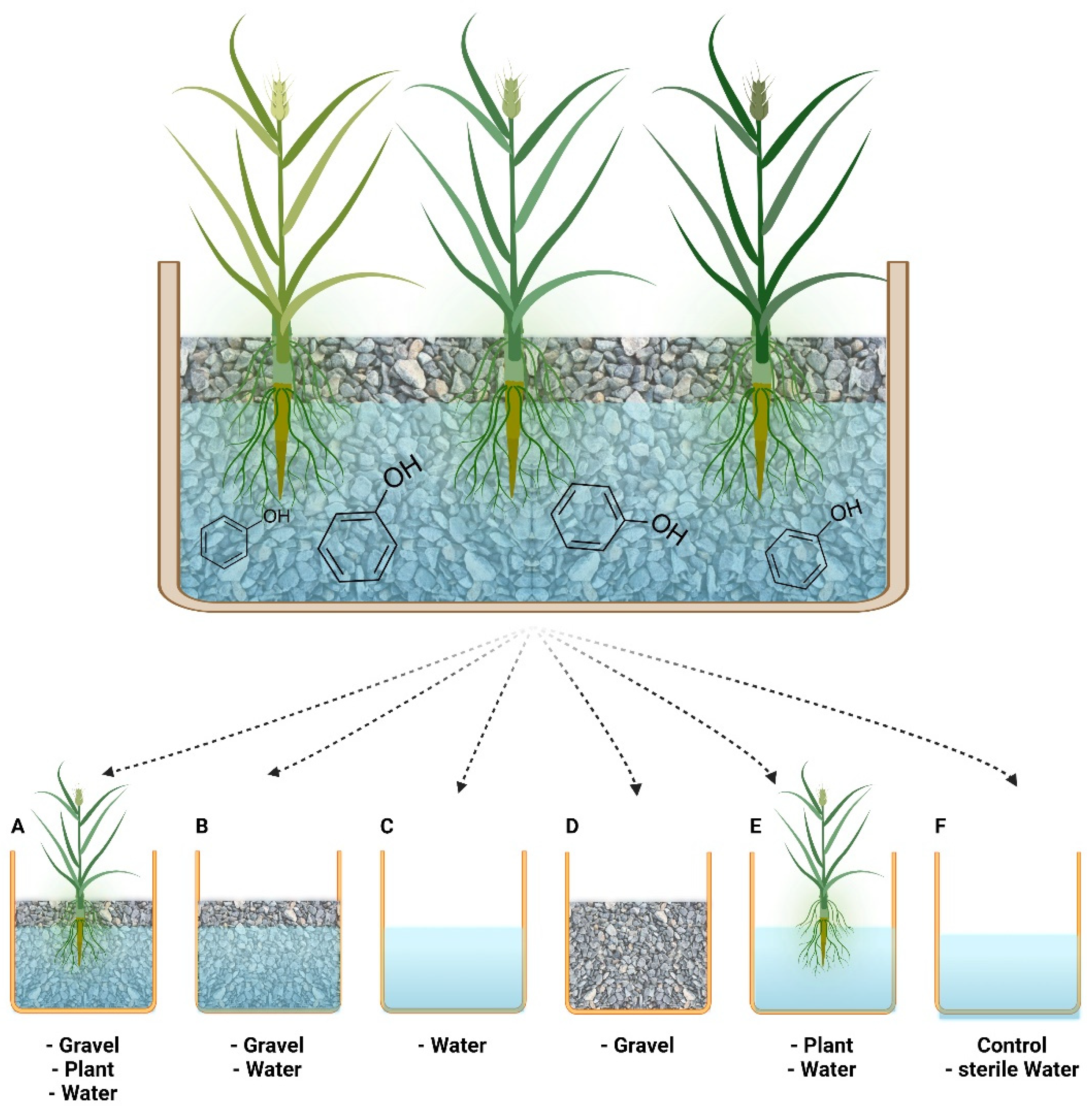
Figure 1. Schematic illustration of the different components of a sub-surface flow CW. Bottom: Illustration of an experimental procedure in which constructed wetland system components are transferred to different tanks in order to monitor the partial contribution of each component to the overall purification process. This includes the following treatments: (A) gravel, plant, water; (B) gravel, water; (C) water; (D) gravel; (E) plant; (F) control (sterile water). The figure was created with BioRender.com. (accessed on 14 February 2022).
Here will focus on the contributions of the three main components—plants, porous bed, and microorganisms—to the total removal of phenol (a model industrial contaminant) in subsurface flow CWs. Phenol (C
6H
5OH) forms the main building block of polycyclic aromatic hydrocarbons (PAHs), which have both carcinogenic and mutagenic properties, and thus pose a serious threat to the health of both humans and the environment. According to Zhao et al.
[12], the use and efficacy of CWs for removal of PAH from effluent has yet to be analyzed in depth, despite the higher cost effectiveness and environmentally friendly nature of this approach with respect to other methods. Degradation of PAH produces phenol
[13][14]; the ease of monitoring phenol concentrations in the influent and effluent of CW systems makes such systems an attractive choice for phenol removal. This has been shown by a number of studies
[15][16][17][18][19][20], which reported total or high (90%) removal of phenols from water through a process of mineralization in CWs.
In general, the purification processes in CWs include biodegradation, filtration, sorption, plant uptake, and sedimentation. Although the partial significance of each process to the overall purification depends on the characteristics of the pollutant, the adsorption and volatilization of phenol have been shown to be negligible compared to the biological degradation
[21][22]. Furthermore, studies suggested that the extent of plant uptake contribution to the overall removal of phenol is insignificant, and indicated that the major role of plants and their root system is to facilitate microbial degradation by providing a suitable habitat for biofilm growth
[22][23].
2. The Partial Contribution of Plants to Phenol Removal
Previous research has demonstrated phenol removal by plants at different rates
[24] and using different plant species, including soybean
[25], alfalfa
[26], and willow
[27]. It is unclear whether sterility was maintained in all studies, thus it cannot be unequivocally stated that phenol removal was always performed by the plants alone. Indeed, a study by Kurzbaum et al.
[28], using non-sterile vs. sterile plants (antibiotic supplementation to prevent rhizospheric bacterial activity), showed that plants alone contribute very little to overall phenol removal, compared with the native bacterial population.
Studies have shown that plant roots act not only as attachment substrates, but also provide a favorable environment for root-attached microorganisms, supplying important biological exudates, including amino acids, simple sugars, complex carbohydrates, and oxygen
[8][29][30]. Few of these studies have explored the favorable environment provided by plant roots in CW systems, in comparison with inert surfaces (e.g., porous bed, gravel etc.). In particular, the magnitude of this “rhizosphere effect” is unclear, considering the continuous movement of the wastewater, which dilutes the exudates and washes them away from the roots. Thus, it is not clear to what extent the plant–microbial interaction accelerates biodegradation in comparison to the porous bed-microbial interaction. Many studies have shown that macrophytes (i.e., plants) have a positive effect on contaminant removal in CW systems
[31][32][33], although whether this beneficial effect results from the higher surface area of the plant roots or the supply of essential nutrients for microbial metabolism has yet to be proven. Other studies have claimed that plants actually absorb and adsorb some of the phenols and other organic contaminants directly into their own tissues
[34], although Kurzbaum et al.
[35] found that the plant contribution alone (using sterile plants) contributes a negligible part of pollutant removal compared to the CW bacterial community.
One of the most significant components of a wetland system is the plants that it supports. There is a broad consensus that the overall contribution of plants to wastewater treatments in CWs is beneficial, but expertise for designing and maintaining plantations is still lacking
[36]. Moreover, there is no consensus regarding the ways plants promote effective water treatment in CWs. The benefit of plants to CWs depends primarily on the type of CW—its direction (horizontal or vertical) and type of flow (surface or subsurface), and the presence or absence of recirculation. Other factors, such as wastewater quantity and quality, treatment medium, plant species composition, plant management, and climate, are also significant.
Planted wetlands have been shown to effectively remove pollutants with no adverse effects on the plants themselves. In their study on CW systems, Polprasert et al.
[16] used cattail (
Typha) species to remove phenols and other organic compounds. Regarding phenol removal, here clarifies the importance of plants for phenol degradation through the large habitat provided by the roots to the microbial biofilm, rather than through the adsorption, absorption and phytodegradation of the phenols in the treated water.
3. The Partial Contribution of Planktonic Bacteria and Gravel/Root Biofilms to Phenol Removal
Biodegradation of phenols by microorganisms in CW systems occurs through aerobic and anaerobic microorganisms
[18]. Although many studies refer to CW systems treating sewage mostly as anaerobic, Stottmeister et al.
[8] suggested a thin layer of aerobic conditions on the root surface causing some oxygen release from the helophytes’ roots.
Under aerobic conditions, oxygen is used as an electron acceptor in the phenol biodegradation process by various species of bacteria and fungi
[21]. Phenol-degrading aerobic bacteria can transform phenol into intermediates that enter the tricarboxylic acid cycle through ortho- or meta-pathways of degradation. The first step in both degradation pathways is monohydroxylation at the o-position of the aromatic ring by the enzyme monooxygenase: phenol hydroxylase. Anaerobic phenol degradation occurs under nitrate-reducing, sulfate-reducing, methanogenic, and iron-reducing conditions. The studies by Sikkema et al.
[37] and Krastanov et al.
[38] describe phenol degradation by yeasts and filamentous fungi as an ortho-mechanism (3-oxoadipate pathway) whose intermediates enter the central metabolism as succinate and acetyl-S-CoA.
The various bacterial populations in CW systems have been investigated to a limited extent. Gagnon et al.
[39] explored the effect of plant presence on the density and activity of the microorganisms, while Pollard et al.
[40] studied how emergent plants are affected by stem-attached biofilms. Toyama et al.
[41] investigated the biodegradation performance of both rhizosphere and suspended bacterial populations, while Collins et al.
[42] compared the water treatment capabilities of CW mesocosms using real plants, plastic plants, or no plants, alongside the bacterial communities. Plant roots and the porous bed were both shown to be significant for bacterial colonization; however, these studies do not allow these two surfaces and their activity to be directly compared in CW systems. Based on current knowledge, it would assume that biofilm bacteria, having greater mass, would contribute more significantly than planktonic bacteria to removal of organic contaminants
[40][43].
4. Conclusions
Phenol degradation by the main components of subsurface flow CW systems—porous bed, plant root, and bacteria—has been described in detail here. Furthermore, the effect of the organic matter content of the water on the growth and performance of the different bacterial components—root-attached biofilm, gravel-attached biofilm, and planktonic bacteria—has been detailed. Although many studies have focused on describing and monitoring the contribution of CW system components to the purification process, few have focused on describing the partial contribution of the three bacterial groups (namely root-attached, gravel-attached, and planktonic) to pollutant degradation. Additional research is required to elucidate the interactions among plants, microorganisms, and the porous media, particularly with respect to any synergistic or antagonistic effects. One direction for future research could be the contribution of the rhizodeposition effect to the degradation of recalcitrant organics (as a source of co-metabolites and catalysts for enzymatic processes). Another research direction could be the addition of attachment surfaces (such as fibers, wood chips etc.) to the CW in addition to the gravel and plants, to increase biofilm amounts and niches in order to reduce the HRT needed for efficient treatment. Finally, research on successful bioaugmentation processes for degrading specific pollutants in CW systems will open up new opportunities in this field, especially with respect to industrial wastewater and wastewater rich in micropollutants. Water purification by CW systems can be improved once scientists better understand the underlying mechanisms of purification by root-attached, gravel-attached, and planktonic bacteria.