Electrospun scaffolds are an important focus of regenerative tissue engineering due to their extremely thin fibers with large surface areas, superior mechanical properties, and ease of processing. Studies to design, fabricate and characterize fibrous scaffolds have been manifold. The characterization of morphology is essential to the main purpose of such scaffolds: to aid in cell proliferation.
1. Physical Methods
1.1. Gas Pycnometry
Gas (commonly helium) pycnometry is a method to measure the volume of solids, based on Boyle’s law, also known as Boyle–Mariotte’s law, and is shown in Equation:
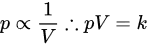
It states that the product of pressure
p and volume
V is some constant
k. The most basic setup for this approach requires a reference chamber of a known volume connected by a valve to a sample chamber, and a manometer associated to each chamber. First, the pressure of both chambers is measured. Then, the valve connecting the chambers is opened, allowing the pressure to reach an equilibrium. This setup is shown in
Figure 1a, where p1C is the initial pressure of the chamber containing the sample, V1 is the gas volume in the chamber, V2 is the volume of the reference chamber, p2C the initial pressure of the reference chamber, and pO is the equilibrium pressure reached after the valve is opened. The volume of the sample can be calculated applying Boyle’s law.
Figure 1. Schematic overview of physical methods for scaffold characterization: (a) Gas pycnometry. (b) Mercury or liquid intrusion. (c) Liquid extrusion. (d) Capillary flow. (e) Liquid displacement. (f) Liquid pycnometry. (g) Apparent density method. (h) Apparent volume method. (i) Gas adsorption. (j) Permeability method. Illustrations created with Inkscape.
Gas pycnometry allows quantitative assessment of scaffold porosity. The approach to convert the volume to porosity is to measure the apparent volume of a cube that the scaffold has been cut into, using a caliper. The measured pycnometer volume is then inverted and divided by the apparent volume, the result being porosity
[39][1], as shown in Equation:
Inter- and intra-fiber porosity can be established using He-pycnometry, the results of which are exceptionally accurate to the extent that they account for much of the void space that is unusable for cell infiltration
[40][2]. However, it is often designated as the gold standard for porosity measurement of solids. It does not contain issues with surface hydrophobicity, chemical reactivity and does not damage the structure
[41][3]. Closed pores cannot be characterized by gas pycnometry, but there are virtually no closed pores in an electrospun scaffold. The method has been used multiple times along mercury intrusion porosimetry
[42,43][4][5], SEM
[44][6], or gravimetry
[45][7] to comprehensively characterize pores. It has also been seldom used to characterize only the density of an electrospun scaffold
[46][8].
1.2. Mercury Intrusion Porosimetry
Mercury intrusion porosimetry (MIP) is a method in which mercury is pressured into a porous solid. A basic setup is depicted in
Figure 1b. As a non-wetting liquid, mercury does not intrude microscopic pores by capillary action. The required external pressure
p is directly related to the surface tension
γ
and the diameter of the pore
D, as well as the contact angle of mercury
θ—commonly approximated at 140°, according to Washburn’s equation
[47][9], adapted for the method performed in a vacuum, as shown in Equation:
Many morphological properties can be obtained from this method
[34][10], including pore volume, pore size distribution, pore tortuosity and pore/throat ratios. This is performed by not only measuring the intrusion curves, but also the extrusion, providing information about the shape. The size of pores that can be characterized are within the 30 nm–0.2 mm range
[48][11].
It was found that for some micro-scale scaffolds, mercury porosimetry could not provide results due to the potential deformation of the scaffold, caused by the required high pressures
[49][12]. Correction algorithms were developed to improve measurements in structures with pore sizes <10 µm
[50][13]. This was used to characterize scaffolds successfully
[51][14]. MIP has commonly been used to determine porosity and pore size distribution
[52][15], as well as only pore size
[53][16].
Although the results of mercury porosimetry are applicable only to a cylindrical shaped pore, it is assumed that an effective cylindrical pore diameter is being measured when the pores have other shapes
[54][17]. Inkbottle shaped pores (a type of blind pore) might make this assumption an issue
[55][18].
1.3. Liquid Intrusion Porosimetry
Liquid intrusion porosimetry is equivalent to MIP, with the exception that other non-wetting liquids such as oil or water are used. Due to the lower viscosity of the liquids applied here and the pressures needed, smaller pores may be measured, as small as 1 nm
[48][11].
1.4. Liquid Extrusion Porosimetry
Liquid extrusion porosimetry (LEP) is a method in which a liquid is pushed out of a porous structure by gas. The liquid/gas interface can be seen in
Figure 1c. The relationship between the differential pressure, pore diameter
D, contact angle of the liquid
θ and surface tension
γ is described by the Young–Laplace equation
[56][19], Equation.

Moreover, the technique has been used to derive a distribution function
fV for the pore volume and to calculate the resulting porosity by integrating in all the pore diameter range according to
[57][20]. This is shown in Equation.
This method was used to describe pore volume, pore volume distribution, pore shape and specific surface area, but is only applicable to through pores and will not provide proper measurements for blind pores
[56][19]. It can characterize pores in the size range 100 nm–2 mm
[48][11]. This technique assumes for all purposes that pores are cylindrical
[54][17] or spherical
[58][21]. In the examples mentioned above
[54[17][19][21],
56,58], the fluorocarbon Galwick was used as a liquid agent.
1.5. Capillary Flow Porometry (Extrusion Flow Porometry)
Capillary flow porometry (CFP) is similar to LEP, in that gas is used to push liquid out of the structure and the Young–Laplace equation is used. However, the pressure is only increased slowly so as to reach the “bubble point”, at which the largest pores are freed from liquid (see
Figure 1d). It is then further gradually increased until the structure is completely dry. The resulting pressure/flow rate curves can be interpreted to provide information on pore throat diameter, pore shape and surface area, but it only measures through pores
[56][19]. However, other researchers found that it was only capable of assessing pore throat size
[59][22]. It can characterize pores of sizes 13 nm–0.5 mm
[48][11].
Moreover, it was reported that deformations of nonwovens produced by the low pressures used in CFP are not significant and not cumulative
[60][23]. The results of CFP, combined with other methods such as LEP, show that pore throat size, pore size and pore diameter increase with increasing fiber width
[54][17]. CFP has been used for providing accurate results in measurements of porosity
[61][24]. It was applied to characterize average pore sizes or their distributions on many occasions
[62,63,64,65,66][25][26][27][28][29]. It presents issues when characterizing inkbottle shaped (also known as bottleneck) pores
[67][30], however, these are rarely found in electrospun scaffolds.
1.6. Liquid Displacement Method
Liquid displacement is a method often used to characterize scaffold porosity
[68,69,70][31][32][33]. In the liquid displacement method, the scaffold is added to a known volume of liquid, commonly ethanol, and is often assisted by various techniques to ensure that all pores are completely filled with liquid. The resulting volume
V2 is measured, and the impregnated scaffold is removed from the container. The amount of liquid lost to the impregnation of the scaffold
V3 is recorded, and this volume is equivalent to the void volume. From these measurements, porosity can be calculated according to Equation
[71][34].
This method was also used with hexane as a displacement liquid due to ethanol potentially shrinking the silk scaffold
[72][35]. However, ethanol was previously used on silk scaffolds and the
resea
uthorchers did not report shrinkage
[73][36]. Some research indicates that ethanol affects silk structures
[74][37]. The method is schematically depicted in
Figure 2e.
1.7. Liquid Pycnometry (Archimedes’ Principle)
Liquid pycnometry follows the same steps as the liquid displacement method, with the exception that instead of measuring volumes, weights are measured. The whole procedure takes place in a pycnometer filled with a liquid. First, the weight of the liquid and the dry weight of the scaffold
ws are measured, then the scaffold is inserted into the liquid, and vacuum or other methods are used to ensure the impregnation of the scaffold. Once this has been achieved, more liquid is added to compensate for the liquid initially displaced by the scaffold until the pycnometer is full again. The weight of liquid and scaffold together
w2 is recorded, and then that of leftover liquid when the impregnated scaffold has been extracted .
Figure 1f shows a slightly different method which is also reported in the literature, the difference being that not only a pycnometer, but any container can be used. Independent of the liquid density, the porosity can be readily calculated
[75,76,77,78,79,80][38][39][40][41][42][43] using Equation.
1.8. Apparent Density Method (Gravimetry)
In this method, the scaffold is cut into a cube, then its apparent volume
V is determined with a caliper. The scaffold’s mass
m is subsequently measured (see
Figure 1g), and the resulting apparent density is calculated and compared with the density of the polymer, providing the porosity value
[81[44][45][46],
82,83], according to Equation.
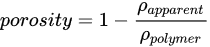
This method can be successfully used to determine porosity if the density of the polymer is known, however, identifying the density of an electrospun polymer can be challenging
[54][17] and was reported to be different from that of the bulk material
[84][47]. Moreover, the soft, fine polymers are prone to deformation during caliper measurement. This issue was not tackled in reported studies, instead only the bulk density of the polymer was used
[81,82,83,85][44][45][46][48]. In some studies, accurate results were produced
[49][12].
1.9. Apparent Volume Method
The apparent volume method is almost the same as the liquid displacement method, except that the scaffold is cut into a cube and its volume is determined by caliper measurements (
Figure 1h). The scaffold is then introduced into a known volume of liquid, after which vacuum pumping or other methods to facilitate impregnation are performed. The final volume is recorded, allowing for a slightly different technique to measure porosity
[81][44], calculated by Equation.
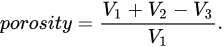
1.10. Gas Adsorption (BET, BJH)
The Brunauer–Emmett–Teller (BET) Theory
[86][49] lays the foundations for a method in which a gas, commonly N
2, adsorbs to the surface of a measured solid and provides a quantitative assessment of its specific surface area. The data acquired by BET measurements have the form of isotherm curves—with standards described in IUPAC
[87][50] providing information on each adsorption layer. These adsorption layers are schematically shown in
Figure 1i, where it is possible to see how a single layer of atoms or molecules adheres to the surface, followed by subsequent layers as described by the so-called Frank–van der Merwe growth
[88,89][51][52]. Each of these layers has a respective adsorption enthalpy.
The resolution of this method is extremely high, since it can characterize the surface area of pores as small as 0.5 nm (but only as large as 2 µm)
[48][11]. The method is sometimes used to determine specific surface area
[90,91[53][54][55],
92], only porosity
[93][56], and sometimes to characterize porosity, mean pore size, and pore size distribution
[94,95,96,97,98][57][58][59][60][61]. For the purpose of determining pore size and distribution, the Barrett–Joyner–Halenda (BJH) method
[99][62] is used. BET measurement, on the other hand, is known to be associated with great uncertainty
[100][63].
1.11. Permeability Method
In the permeability method, a liquid is pressed through a solid sample as seen in
Figure 1j. The permeability method uses measured permeability by Darcy’s Law
[101][64]. The relationship is described in Equation, where
τ is the permeability,
Q the volume per time unit,
η the viscosity,
h the length of the fluid column,
F the cross-sectional area perpendicular to the flow,
p the applied pressure, and
t represents time:
This approach is used to acquire fiber diameter and pore size
[101][64]. When used on electrospun scaffolds, this method produced accurate results for the fiber diameter, but not for pore size
[102][65].
2. Imaging Methods
2.1. Scanning Electron Microscopy (SEM)
Scanning electron microscopy is a method in which the surface of a sample is examined, providing information on its morphology. Commonly, to achieve this, an electron beam is directed at the sample, which then excites the atoms on the surface, causing secondary electrons to be emitted. These are then detected, and an image can be constructed. Although there are multiple types of SEM, the most common involves placing the sample in a high vacuum
[103][66]. Samples are often nano sputtered with gold, platinum, or other conductive materials to render the surface conductive and to avoid charging effects. Further preparation steps are often taken in order to ensure the sample is dry and can withstand the low pressure
[104][67]. An advantage of SEM over physical methods is that it can qualitatively assess cell growth on surface layers
[105][68]. The highest attainable resolution of SEM is approximately 1 nm
[106][69].
For 3D scaffolds fabricated by stacking electrospun mats, a method was described that used image processing software on the SEM images to determine porosity accounting for the different layers
[107][70]. This method was later used to determine porosity
[108][71], however, the average pore diameter, fiber diameter, and interconnection of the pores were measured using image processing software, meaning those properties of the surface structure were extrapolated to the 3D composition of the scaffold. Another method to produce 3D scaffolds by alternating microfiber with nanofiber mats was also reported. The SEM-measured characteristics included porosity, fiber diameter, and pore size
[49][12].
SEM has also been used to characterize scaffold porosity by converting images to binary, whereby fibers are black and pores white or vice versa
[109,110][72][73]. In some cases, the fiber diameter was also quantified using image processing software.
There are many instances where SEM has been used to measure fiber diameter only, as well as to qualitatively assess morphology
[40,54,85][2][17][48]. Occasionally, it is also used to determine mean pore sizes
[76,102,111][39][65][74].
One extremely different approach consisted of using a focused ion beam (FIB) to remove surface layer coating and progressively take 2D SEM images of the subjacent layers, allowing for subsequent 3D tomography
[112][75]. It was concluded that the 2D imaging was insufficient in providing insight into the true morphology of the scaffold, however, the 3D method caused results with much noise and was not compared with other 3D characterization methods.
SEM was used to characterize fiber diameter/alignment, pore diameter, and porosity by measuring apparent density from the SEM images and comparing it to the bulk density of the electrospun polymer
[113][76]. A similar method was used later
[114][77], with the exception that porosity was calculated by the physical apparent density method.
Concerns were raised that the electron beam may damage nanometer-scale fibers
[115][78], in addition to the fact that SEM is only capable of characterizing surface properties
[106][69], which might not extend uniformly throughout the scaffold, or even be representative of the interior of an otherwise uniform scaffold. It was reported that the vacuum of the microscope could cause shrinkage of certain samples
[116][79].
2.2. Transmission Electron Microscopy (TEM)
Transmission Electron Microscopy (TEM) is a microscopy technique that is equivalent to light microscopy in many aspects, except it uses electrons instead of photons, i.e., light, thus allowing for a much higher resolution due to the easily achievable small de Broglie wavelengths, in addition to presenting minor differences in functionality
[117][80]. However, the optimal thickness for studied samples is in the low nm range
[118][81], rendering it unsuitable for many scaffolds, and only having the potential to examine individual fibers, however with an excellent resolution of down to 0.2 nm
[106][69]. It has been used on individual fibers, especially for fibers containing nanomaterials
[119][82].
2.3. Atomic Force Microscopy (AFM)
Atomic Force Microscopy (AFM) is a type of microscopy that employs a cantilever probe to physically touch the surfaces, when operated in contact mode. The Coulomb forces exerted on the cantilever by the surface of the sample can then be characterized by piezoelectric components or laser-detector setups. This allows for topographical measurements with resolutions of under 1 nm
[120][83]. When in contact mode, however, the stiffness of the cantilever is superior to that of the bonds present in many polymers. One advantage of AFM is that it allows for the characterization of not only morphological, but of mechanical and even physical–chemical properties.
AFM was previously used to characterize 2D and 3D surface topography. One important aspect to be noted is that the images show a noticeable variation in fiber diameter
[121][84]. It has also been used to assess the nanostructure of the fibers themselves
[122][85].
Moreover, AFM can be operated in non-contact mode, which might prove advantageous since the nanofibers may be sensitive to the forces exerted by the cantilever. This method was reported for electrospun scaffolds
[123][86]. Another possible operation mode is intermittent contact, or “tapping” mode. Often, it is combined with phase imaging, whereby the shift between the phase of the cantilever and the phase of the response oscillation is recorded. The use of phase imaging has also been reported in the literature, and has provided excellent images of the nanostructure on the fibers as can be seen in
[124][87].
As of 2021, AFM is the tool with the highest resolution
[106][69] capable of providing insight into the surface structure of the fibers themselves.
2.4. Micro-Computed Tomography (Micro-CT)
Micro-computed tomography is a technique in which X-rays are used to create a 3D image of the structure of a sample. The pixel has a side size in the µm range. This allows for the characterization of µm-scale structures. It can be used in combination with phase-contrast techniques in order to increase its resolution
[125][88]. A significant advantage of micro-CT is the potential of its use on in vivo samples
[126,127][89][90].
One issue with micro-CT is that the use of higher resolutions leads to smaller scale measurements, which inevitably causes issues when extrapolating the information to the whole structure, especially if a degree of anisotropy is given
[128][91]. The optimal use of micro-CT in tissue engineering, including pixel size, the use of percolation theory and other significant factors has been reported
[129][92].
In this study, however, the criterion for defining the relevance of resolution is the measurement of cells. Considering that the texture of the fibers themselves bear an influence on cell attachment [31], it can be argued that the resolution is insufficient for full morphological characterization.
It was often found that for electrospun nanofibers, the resolution of micro-CT, down to 1–3 µm, was insufficient [54]. In this study, a further difficulty was reported, which was the assessment of a threshold for image processing to identify fibers and pores.
2.5. Nano-Computed Tomography (Nano-CT)
Nano-CT uses the same technology as micro-CT, with the pixel sizes being in the nm range
[125][88]. It is commonly used in combination with phase-contrast technology. Resolutions better than 50 nm were already being reported in 2007
[130][93], making it a tool capable of characterizing many features of nanoscale scaffolds
[131][94], however, many research institutions lack access to nano-CT equipment.
Zernike phase contrast nano-CT was effectively used to fully characterize the morphology of electrospun scaffolds
[40][2], and it has even been used to characterize cell growth on such scaffolds successfully
[132,133,134][95][96][97]. The effect of how essential thresholding is to images created by nano-CT can be seen in
[135][98], although only fiber alignment was qualitatively assessed from this image.
2.6. Confocal Laser Scanning Microscopy (CLSM)
Confocal laser scanning microscopy (CLSM) is a technique that allows for spatial filtering and increased resolution of light microscopy through the suppression of light not transmitted directly from the focused part of the sample. It can be combined with fluorescence microscopy and is therefore another powerful tool to image cell growth. Due to the ability of CLSM of obtaining 2D images at different depths, a 3D image can be reconstructed
[136][99]. The resolution of CLSM is about 10 nm on the XY-plane and about 800 nm on the
Z-axis. It can penetrate samples up to 0.3 mm
[106][69].
The use of CLSM to characterize porous scaffolds was first reported in 2004
[59][22], but it was used without treating the polymer so as to make it fluorescent. The resulting images were diffuse and unclear, although the
resea
uthorchers believed they characterized the scaffold structure better than cryo-SEM. However, due to the diffuse nature of the image it was difficult to extract pore sizes.
In 2013, a novel method of incorporating Quantum Dots—fluorescent nanoparticles—into the polymer solution, along with image post-processing, was introduced
[61][24]. The results mostly coincided with the experimentally and theoretically predicted ones, with fiber volume, total pore volume, porosity, interconnectivity, structure thickness, and average pore throat diameter being measured.