The natural human telomeric G-quadruplex (G4) sequence d(GGGTTAGGGTTAGGGTTAGGG) HT21 was extensively utilized as a G4 DNA-based catalytic system for enantioselective reactions. Modified oligonucleotides (ODNs) based on this sequence were investigated to evaluate their performances as DNA catalysts in an enantioselective sulfoxidation reaction of thioanisole. The HT21 derivative containing an AL residue in the first loop sequence significantly proved to be capable of producing about 84% enantiomeric excess.
1. Introduction
DNA-based asymmetric catalysis has recently attracted increasing attention, thus becoming a particularly interesting tool for organic chemical synthesis. The double-stranded DNA (dsDNA) has been regarded as a catalytic species and applied in various key asymmetric reactions
[1,2,3,4][1][2][3][4]. The right-handed chiral conformation of dsDNA plays a key role in enantioselective catalysis, making this secondary structure a promising chiral inducer
[1,2,3,4][1][2][3][4]. A DNA-based catalyst comprises a non-chiral ligand that can chelate a transition metal ion; therefore the catalyzed reaction takes place in, or very close to, the DNA helix to allow the chirality of DNA to be transferred onto the reaction
[1,2,3,4][1][2][3][4]. The DNA double helix provides the chiral microenvironment to selectively form one enantiomer of a given reaction product. Several studies have been carried out to highlight the mechanisms by which the chirality is transferred and the influence of the interaction between DNA and the metallic co-factor on the selectivity
[5]. Furthermore, taking advantage of the unique structure of L-DNA, left-helical enantioselective induction has been obtained in different DNA-based asymmetric catalyzed reactions, thus allowing tuning the absolute configuration in DNA-based asymmetric catalysis, predicting access to both enantiomers for any reaction
[6].
Recently, G-quadruplex DNA structures (G4 DNA) met with growing success in catalysis, with these structures able to induce sensible levels of enantioselectivity in several asymmetric reactions. Indeed, the concept of G4 DNA-based catalysts was first introduced in 2010
[7]. Since then, G4 DNA have been applied in asymmetric Diels–Alder and Friedel–Crafts reactions
[8,9][8][9]. Furthermore, G4 DNA were employed to speed up the transformation in aldol reaction
[10] and sulfoxidation
[11], confirming that the G4 DNA could also act as a suitable chiral template.
Unlike dsDNA, G4 DNA possess a remarkable structural variability, depending on the strand molecularity (tetra-, bi-, monomolecular) or arrangement (parallel, antiparallel, etc.), the presence and type of the loops (propeller, lateral, diagonal), the glycosidic conformation (
syn or
anti) of the G-residues, and the groove sizes
[12]. Furthermore, the conformation of G4 DNA can be self-regulated by the external environment. For example, the human telomeric G-quadruplex sequence d(GGGTTAGGGTTAGGGTTAGGG) HT21 can form anti-parallel, parallel, or hybrid-type monomolecular G-quadruplex conformations in different buffer solutions
[14,15][13][14] (
Figure 1). The remarkable structural variability of G4 DNA constitutes a valuable resource to design new DNA-based catalysts, usable in synthetic organic chemistry. In turn, the study of the relationship between DNA structure topology and catalytic reaction performances could be useful to recognize and clarify the different features that influence the stereo-induction.
Figure 1. Schematic representation of G-quadruplex topologies formed by HT21 in different buffer solutions.
The catalytic performance of the G-quadruplexes can be influenced by several factors, among them, the topology of the quadruplex and the nature of the ligand, which can be non-covalently or covalently linked to the secondary structure, thus tuning the stereoselectivity in DNA-based asymmetric catalysis
[16,17][15][16].
The natural telomeric G-quadruplex HT21 has been extensively utilized as G4 DNA-based catalytic system for enantioselective Diels–Alder
[8,18,19,20][8][17][18][19] reactions, while, to the best of
ourthe knowledge, few studies have been reported on DNA-based enantioselective sulfoxidation reaction
[11,21][11][20]. Enantiomerically pure sulfoxides have interesting potentials both in pharmaceutics, as biologically active compounds
[22][21], and in asymmetric synthesis, as chiral ligands and organocatalysts
[23][22]. The main method to obtain enantiopure sulfoxides is the enantioselective oxidation of sulfide. Most of the biological oxidation reactions are usually catalyzed by protein enzymes and cofactors in water
[23,24][22][23]. The first DNA-based enantioselective sulfoxidation reaction was achieved in 2016
[11] with an achiral Cu(II)-4,4′-bimethyl-2,2′-bipyridine complex non-covalently interacting with G4 HT21, using H
2O
2 as oxidizing agent. The authors found that the G-quadruplex helix backbone is responsible for the catalytic enantioselectivity and activity; indeed, the chirality of DNA can be transferred into the sulfoxides, providing up to 77% enantiomeric excess. A later study, aimed to characterize the interactions of Cu(II)-bipyridine cofactors and thioanisole substrates with HT21, proved that bipyridine complexes showed nonspecific binding properties and the thioanisole interacted with G-quadruplex structure at around the second loop and 3′-end, confirming that the reaction usually occurs on a terminal G-tetrad in a space surrounded by the loop residues
[21][20]. It is generally known that a human telomeric G4 DNA metalloenzyme is able to influence the configuration and the enantioselectivity of the reaction products and that the loop sequence in the G4 DNA catalyst has a high influence on chiral expression
[9].
In order to explore the role of the residues in the loops and to improve the performances of G4 DNA catalysts, a series of HT21 analogues were prepared, in which each sequence contained a chemically modified monomer replacing, one at a time, natural adenosines in the TTA loops. Since minor changes in the loop sequences of G4 DNA can have notable consequences on the chiral orientation of the enantioselective reactions
[9], straightforward chemical modifications were made to single adenosine loop residues in close proximity to the upper or lower G-tetrad, by using commercially available adenosine derivatives (
Figure 2). Specifically, HT21 derivatives containing 8-bromo-2′-deoxyadenosine (A
Br), 8-oxo-2′-deoxyadenosine (A
oxo), and β-L-2′-deoxyadenosine (A
L) were prepared (
Table 1), and the effect of different loop sequences on the activity and the enantioselectivity of G4 DNA metalloenzyme in the sulfoxidation reaction were tested. These modified nucleosides were chosen either to evaluate the electronic and steric effects of different groups in the C-8 position of loop adenines being part of the microenvironment surrounding and orienting the substrate, or to take advantage of an L-DNA residue to transfer chirality and modulate the enantioselectivity.
Figure 2.
Chemical structures of the adenosine derivatives introduced in different loop positions of HT21.
Table 1. Sequences investigated and their melting temperatures.
Name |
Sequence (5′-3′) |
Tm °C (±1) |
HT21 |
GGGTTAGGGTTAGGGTTAGGG |
69 |
HT21-ABr1 |
GGGTTA | Br | GGGTTAGGGTTAGGG |
70 |
HT21-ABr2 |
GGGTTAGGGTTA | Br | GGGTTAGGG |
72 |
HT21-ABr3 |
GGGTTAGGGTTAGGGTTA | Br | GGG |
71 |
HT21-AL1 |
GGGTTA | L | GGGTTAGGGTTAGGG |
73 |
HT21-AL2 |
GGGTTAGGGTTA | L | GGGTTAGGG |
74 |
HT21-AL3 |
GGGTTAGGGTTAGGGTTA | L | GGG |
68 |
HT21-Aoxo1 |
GGGTTA | oxo | GGGTTAGGGTTAGGG |
68 |
HT21-Aoxo2 |
GGGTTAGGGTTA | oxo | GGGTTAGGG |
68 |
HT21-Aoxo3 |
GGGTTAGGGTTAGGGTTA | oxo | GGG |
68 |
2. CD Spectroscopy
CD spectra of the modified HT21 were acquired and compared with their natural counterpart in the same buffer and temperature conditions of the reaction (see below).
The CD profile of natural telomeric sequence HT21 showed a positive peak around 290 nm, a major shoulder at 270 nm, and a smaller one at 250 nm with a small negative peak around 240 nm, accordingly with hybrid-type telomeric G-quadruplex structures
[27][24]. Regarding HT21-A
Br-modified sequences, HT21-ABr1 and HT21-ABr2 displayed CD profiles very similar to each other and to their natural counterpart, apart from slight differences in band intensity around 290 nm, thus indicating that the ability to adopt hybrid-type G-quadruplexes is preserved. More significant differences were observed for HT21-ABr3 CD spectrum in the shoulder region around 250–270 nm, thus suggesting the presence of other G-quadruplex conformations in addition to the hybrid ones (
Figure 3A).
Figure 3. CD spectra of HT21 and its ABr (Panel A), AL (Panel B) and Aoxo (Panel C) derivatives in 20 mM MOPS buffer (pH 7.0) containing 150 mM KCl (20 μM in ODN).
Concerning the spectra of the HT21-A
L-modified G4, their CD profiles confirmed a strong positive peak around 290 nm, peculiar of the hybrid-type telomeric G-quadruplex
[27][24], and a minor positive one at 250 nm, while the shoulder around 270 nm was less marked or completely absent (HT21-AL2) (
Figure 3B).
Finally, HT21-Aoxo derivatives showed CD profiles almost superimposable to each other, particularly HT21-Aoxo1 and HT21-Aoxo2, and closely comparable to that of the natural one, apart from the region around 270 nm, as in some previous cases (Figure 3C).
The whole of the data clearly indicated that the hybrid-type G-quadruplex of the natural telomeric sequence was very likely to be the main conformation adopted by them, despite tiny structural dissimilarities supposedly involving the modified loop regions.
3. Catalytic Properties of HT21 Analogues
To investigate the catalytic properties of HT21 derivatives, the enantioselective sulfoxidation reaction, catalyzed by human telomeric G4 DNA and Cu(II) complexed with 4,4′-bimethyl-2,2′-bipyridine (CuL), using H2O2 as the oxidant, was carried out on thioanisole giving sulfoxide enantiomers. The reaction conditions used, including molar ratio between G4 DNA catalyst and CuL cofactor, potassium concentration, buffer pH, and temperature, were those optimized by Mingpan Cheng et al. [11]. In these conditions ([G4 DNA]/[CuL] = 1:5, [KCl] = 150 mM, pH 7.0, 15 °C), the unmodified telomeric G4 DNA catalyzed the reaction with a full conversion and 56% enantioselective excess (ee) [11].
All the modified HT21 catalysts, except HT21-ABr1 (93% of conversion), complexed with CuL, exhibited conversion data very similar to the natural one, thus indicating that, in general, the introduction of an unnatural adenosine in the HT21 loop sequence did not reduce the catalytic activities of the evaluated modified G4 DNA. However, data clearly indicated that the presence of modified residues significantly affected the enantioselectivities of the G4-based catalysts (Table 2). Indeed, most of HT21-modified sequences revealed minor enantioselectivities (range of 14–37% ee) compared to the natural one, except in the cases of HT21-AL1 and HT21-AL2. Interestingly, while HT21-AL2 proved able to give an enantiomeric excess (54%) strictly comparable to the unmodified sequence, HT21-AL1 exhibited a remarkably improved enantioselectivity (about 84% ee). This ee value was significantly much higher than that of natural G4 DNA catalyst and, to the best of ourthe knowledge, corresponded to the highest enantioselectivity for DNA based oxidation reaction obtained to date.
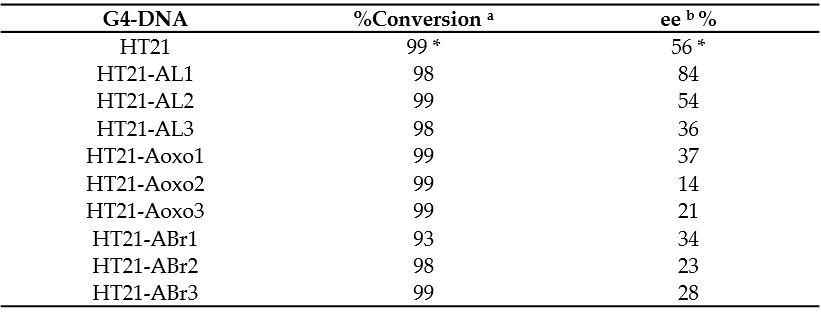
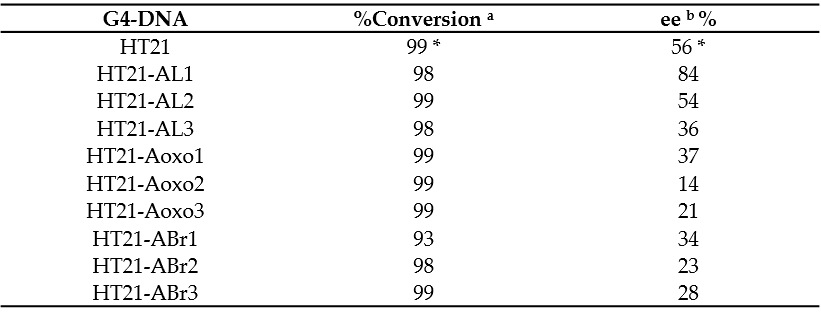
Table 2. Enantioselective oxidation of thioanisole (1). * As reported by Mingpan Cheng et al. [11]. All data are repeated for two separate experiments. a Determined by chiral-phase HPLC within reproducibility of 2%. b Determined by chiral-phase HPLC within reproducibility of 3%.
G4-DNA
|
%Conversion a
|
ee b %
|
HT21
|
99 *
|
56 *
|
HT21-AL1
|
98
|
84
|
HT21-AL2
|
99
|
54
|
HT21-AL3
|
98
|
36
|
HT21-Aoxo1
|
99
|
37
|
HT21-Aoxo2
|
99
|
14
|
HT21-Aoxo3
|
99
|
21
|
HT21-ABr1
|
93
|
34
|
HT21-ABr2
|
98
|
23
|
HT21-ABr3
|
99
|
28
|
G4-DNA |
%Conversion ab |
ee b % |
HT21 |
99 * |
56 * |
HT21-AL1 |
98 |
84 |
HT21-AL2 |
99 |
54 |
HT21-AL3 |
98 |
36 |
HT21-Aoxo1 |
99 |
37 |
HT21-Aoxo2 |
99 |
14 |
HT21-Aoxo3 |
99 |
21 |
HT21-ABr1 |
93 |
34 |
HT21-ABr2 |
98 |
23 |
HT21-ABr3 |
99 |
28 |
These data suggest that, in agreement with those reported for other reactions [9], the three-dimensional structure of HT21, generally preserved in all modified HT21 analogues as indicated by the close similarity of their CD spectra, ensured the notable catalytic performances, giving nearly quantitative conversion. On the other hand, the enantioselectivity of the product largely depended on the loop sequence of the G4 DNA catalyst. Minor modifications in the G4 DNA loop sequence, as the substitution of an A residue with an ABr or Aoxo, significantly reduced the chiral expression of sulfoxidation reaction. However, the replacement of a natural D-residue with a β-L-2′-deoxyadenosine in a specific loop was proven to be useful to increase the ee value of the reaction, thus improving chiral selectivity. OAurthors data confirmed the key role of the loop regions in close proximity to the terminal G-tetrads for chiral induction of the reactions and expanded the G4 DNA catalytic properties in asymmetric oxidations.