1. Introduction
1. Transport Mechanisms Different from Those in Polymers
The commonly accepted “solution-diffusion” mechanism for the permeation of gas species through dense polymeric membranes (P = D × S) [110] can be modified by the presence of the fillers within the matrix. The filler loading can affect the individual permeation terms or both, depending on its properties and on the interplay between the polymeric matric and the nanoparticles. Facilitated transport can occur in the presence of specific additives as a result of preferential adsorption properties or higher affinity of the filler for specific gases (typically CO2) and additional paths made available in the matrix. In virtue of this, performance above Robeson’s upper-bound is possible in mixed-matrix membranes (MMMs).
Different studies compared the effect of porous and non-porous fillers on the MMMs’ performance [17,18,48].
MMMs loaded with non-porous fillers provide transport pathways through the Pebax’s free volume elements and through the spaces at the interface between the Pebax and the fillers.
Additional pathways are made available by porous fillers. However, partial pore blocking can occur in porous fillers when the polymer chains in part penetrate the channels due to a high affinity between the heterogeneous phases. Accordingly, a decrease in the permeation rate is observed with a moderate gain in selectivity if the resulting available spaces are comparable with the kinetic diameters of the gas molecules.
Porous GO was more effective in increasing the CO2 permeability than non-porous one dispersed in Pebax 2533 [48]. The same behavior was reported when comparing non-porous SiO2 to NaX and ZIF-8 porous nanofillers [17] or for organosilicon nanotubes used as porous or non-porous particles [16].
A comparison of nonporous SiO2 with porous ZIF-8 and NaX showed a small increase in permeability for the large pore zeolite NaX [17]. Instead, the impermeable SiO2 resulted in better permeability values, while the best results were obtained with ZIF-8 fillers. These results were due to a partial blockage of the zeolite pores by the polymer chains due to a good adhesion to the zeolite surface. However, the partially blocked pores of the NaX zeolite acted as molecular sieves and increased the selectivity with respect to neat Pebax or to Pebax/SiO2 MMMs.
However, a partial blockage of the porous fillers by the flexible PE segments of the polymer chain can happen, as reported in the case of MMMs containing MOFs (NH2-MIL-53) [71] and zeolites (DD3R) [60]. A lower CO2 permeability of the MMMs compared to that of the neat polymer was measured for MMMs based on Pebax® 1074 and DD3R zeolite [60]. This behavior depends on a combination of chain rigidification and free-volume reduction in the membranes, and also on the partial pore blockage of the zeolites [60]. A higher loading of DD3R zeolite depresses the permeabilities of CO2 and CH4, while a low loading (1–5 wt%) the zeolite particles enhances the ideal gas selectivity due to an improved in CO2 sorption in the membrane [60].
Two-dimensional-GO loaded in Pebax 1657 blended with PEG-MEA (50 wt%) creates extra diffusional pathways at the interfaces with the polymer causing an increase in CO2 permeability. Interlayer channels between few-layered GO can act as molecular sieve. By increasing the GO content, a region with an optimal combination of CO2 permeability and CO2/N2 selectivity was identified in the range 0.06–0.3 wt%[47].
By analyzing the permeation parameters, it is possible to evidence distinct changes induced by the fillers on the gas diffusivity, on the gas solubility coefficient, or on both permeation terms.
In the case of porous organosilicon nanotubes (PSiNTs), a larger CO2 diffusion coefficient was measured in the MMMs [18]. Indeed, BET adsorption–desorption analysis evidenced the presence of micropores on their walls. Accordingly, in addition to one-dimensional channels of SiNTs, the nanotube walls are an extra transport path for gas molecules in Pebax-PSiNTs.
An increase in the CO2 solubility coefficients was evidenced for Pebax®/PEG-MEA blend membranes incorporating GO nanosheets at up to 0.3 wt%, which resulted in a considerably increased CO2/N2 selectivity [47]. Indeed, GO has a high affinity for CO2 that is enhanced by amino groups [52]. At optimum loading, the amino-functionalized A-prGO creates a tortuous pathway that enhances the permeation of CO2 in the active layer, but hinders the transport to other gases, increasing the CO2 selectivity [52].
ZnCo2O4 enhances the CO2 gas permeability and CO2/CH4 selectivity since the nanosheets provide pore channels that accelerate the gas diffusion and additional interaction sites for CO2 sorption with respect to the neat polymer [28].
A ‘blocking effect’ on CO2 was observed for MMMs containing hydrophilically modified 2D imidazole framework flakes (hZIF-L) [70]. The pore structure of these flakes intensifies the diffusion of gas molecules. However, as the filler content increases, the number of functional groups interacting with CO2 grows, limiting the diffusion of CO2 molecules [70]. Thus, the diffusion selectivity of the MMMs shows an inverse relationship with the hZIF-L amount.
As discussed above, some fillers introduce an additional transport mechanism selectively for CO2. For instance, the thickest 2D nanosheets of ZIF-C MOF bring selective adsorption of CO2, while for the N2, the only mechanism is that of the solution-diffusion model [68]. Instead, the modelling displayed that the addition of N-FLG causes an increase in the diffusivity of N2 (three-fold), while that of CO2 decreases slightly. This was ascribed to the creation of a tunnel for N2 transport by the N-FLG nanolayers, which was confirmed experimentally [44].
Some of the developed MMMs can be categorized as Facilitated Transport membranes since a carrier is used to facilitate the transport of CO2. Amino-functionalized porous nanotubes (N-PSiNTs) in MMMs combine the wall porosity that permits a rapid gas transport, thereby intensifying the diffusion mechanism and increasing the CO2 permeability, with the reversible reaction between amino groups in the fillers, which provides a facilitated transport mechanism that increases the CO2/CH4 selectivity [18]. The amino groups on GO in the case of aminosilane-functionalized graphene oxide (f-GO) nanosheets help to construct a facilitated transport pathway along the polymer–filler interface [53]. A similar role was shown by IL-NH2 moieties on the GO surface, which are able to react reversibly with CO2, and thus, increase the selectivity versus nitrogen and hydrogen as pure gases and in the mixture [50].
Polyethyleneimine (PEI), a solid amine adsorbent for CO2 [111], was used to modify Nanodiamonds, thereby functioning as an interfacial binder and as a CO2 carrier [40].
The enhanced transport of CO2 molecules in Polymer Electrolyte membranes containing KBF4 is due the reversible interaction of the dissociated potassium ions with CO2 [104]. FT-Raman spectroscopy confirmed the dissociation of potassium ions upon thermal treatment of the material.
The facilitated transport mechanism was added to the solution-diffusion mechanism by introducing an aniline carrier to the Pebax matrix, promoting both the permeability and selectivity of CO2. Molecular simulation indicates that aniline molecules can move freely between polymer chains with a high diffusion coefficient, thus enabling the transport of CO2 molecules through ‘two hopping and vehicle’ mechanisms. Therefore, compared to the neat membrane, those loaded with aniline show a CO2 diffusivity that is increased by 7.5 times [7].
In the case of Ionic Liquid-decorated nanocages, the [Hmim][NTf2] on the external surface of LDHN absorbs more CO2 molecules than CH4, thereby improving the selectivity of CO2/CH4 [86]. In addition, [Hmim][NTf2] in the interlamellar spacing of LDNH dissolves the captured CO2 that permeates into LDHN shells. The CO2 transport is accelerated since the dissolved CO2 combines quickly with CO32− carriers that are present in LDHN. Furthermore, the [Hmim][NTf2] within the internal hollow core of the nanocages adsorbs CO2. Therefore, the synergy between the IL and the LDHN significantly increases both the permeability and selectivity of CO2 [86].
The greatly improved CO2 separation performances in the dry state are even better under humidified conditions for MMMs containing aminosilane-functionalized graphene oxide (f-GO) nanosheets [53] and for 2D ZnCo2O4 nanosheets [28]. Under humid conditions, the interlamellar channels of MXene fillers enable fast and selective CO2 transport [83]. In particular, owing to the high upper limit of loading compared to GO, Pebax-MXene membranes show noteworthy performance [83].
In the presence of humidity, water was shown to play a key role in the reversible reaction between CO2 and carboxyl/carbonate groups for LDHs that were intercalated with amino acids (AA-LDHs) [87].
The combination of CaLS and water induce a swelling that is not excessive due to the hydrophobic interaction between CaLS and Pebax, resulting in an optimal FFV and moderate salting-out effect that leads to an extremely high CO2 permeability (3585 Barrer) and good CO2/gas selectivity [103].
In the case of mixed gas testing, competitive sorption phenomena are possible; these can affect the separation performance, as evidenced in a study conducted on ZnCo2O4 2D nanosheets [28]. Lower CO2 permeability and CO2/N2 and CO2/H2 selectivity were measured in mixed gas tests as compared to the corresponding values obtained via pure gas measurements [50].
[1]Various studies showed a good compatibility of the polymers belonging to “Pebax family” with a huge number of fillers for the preparation of nanocomposite membranes to be used in CO
2. Defects—Causes and Countermeasures
The filler dispersion within the polymer and the fabrication of membranes with an ultra-thin defect-free selective layer are the key challenges for the development of MMMs.
A poor adhesion between the heterogeneous phases causes the appearance of some defects at the particle–polymer interface in a composite structure. This aspect is particularly significant in the case of glassy polymers, which have more rigid chains than rubbery polymers.
Defects arise from the creation of void spaces between filler particles and the polymer matrix in the zone surrounding the particle where a rigidification of the polymer chains can happen, depending on the particle/polymer interaction, as discussed above. However, defects also result from the formation of agglomerates. As already discussed, at higher filler concentrations, the probability of defects rises. In addition, a non-uniform filler dispersion negatively influences the membrane performance. The higher density of some particles, with respect to the polymer (e.g., ρ = 3.65 g/cm3 for Al2O3 and ρ = 1.14 g/cm3 for Pebax matrix), causes their sedimentation during the casting procedure [32]. Channeling is a consequence of the non-uniform distribution within the host matrix and agglomeration among the particles. This phenomenon is detrimental in terms of the transport properties of the membrane because of the non-selective preferential paths for the gas molecules. As the filler size decreases, the cohesive forces are stronger, favoring this behavior in the presence of high loadings.
Particle pre-treatment (e.g., priming, functionalization, or insertion/grafting of chemical groups) or the addition of plasticizing agents (e.g., Ionic Liquids) are the main approaches to making the heterogeneous phases within an MMM more compatible. Some methods were developed to modify the external surface of the filler particles, and others were applied to make the polymer chains embedding the filler more flexible. The former approach mainly involved depressing the agglomeration tendency of the particles, and the latter involved lowering the rigidity of the host matrix.
Priming and sonication, adopted to incorporate Graphene nanoplatelets (GNPs) in Pebax (at 0.3, 0.5, 0.7 and 1 wt%), were effective in terms of avoiding the agglomerates below 1 wt% [43].
A good dispersion was reported after the functionalization of MOFs (ZIF-8-NH2 [66] and UiO-66-NH2 [76]) with amino groups since these units can interact with the PA segment in Pebax, thus making the particles more compatible with the polymer. An improved dispersion was also reported for aminosilane-functionalized graphene oxide (f-GO) nanosheets [53].
Grafting with a silane agent (3-aminopropyl-diethoxymethylsilane) and surface modification with carboxymethyl chitosan led to better compatibility between the Titanium oxide nanoparticles and the polymer, better thermal stability of the nanocomposite membranes, and satisfactory dispersion of the nanofillers, as shown via FT-IR, DSC, TGA, and SEM [30].
Poly(ethylene glycols) (PEGs) were used in blends with the Pebax matrix in different works [27,36,47,58,73,92] since they are known to significantly increase the permeability of Pebax [112], while keeping the CO2/N2 selectivity [47]. A plasticizing effect with a significant reduction in the crystallinity of the PA phase was reported upon the addition of PEG-MEA to Pebax 1657 [45]. Indeed, the addition of low molecular weight PEG to Pebax decreased the activation energy of permeation [113]. The contemporary presence of PEG and nanoparticles can also increase the selectivity. The addition of PEG was found to improve the compatibility of NaY and the membrane matrix, significantly enhancing the CO2 permeability and the CO2/N2 selectivity [58].
Another study proposed Pluronic, a non-ionic block copolymer composed of a hydrophilic group of poly(ethylene oxide) (PEO) and a hydrophobic group of poly(propylene oxide) (PPO), to prepare MMMs based on Pebax® 2533 and ZIF-8 [67]. Glycerol was considered as a low molecular weight additive and was found to provide good CO2 affinity [94]. Glycerol alone was effective in enhancing the selectivity but reduced the gas permeability with respect to the neat polymer, while the combination of Glycerol with Cu nanoparticles led to enhanced permeability [94].
Ionic Liquids (ILs) were also exploited as free additives for Pebax [114], resulting in more plasticized matrices with improved permeability. More recently, ILs were introduced in the Pebax MMMs to decorate the fillers before their dispersion within the polymer [50,86,93]. Acidic and basic ILs applied to modify the γ-Al2O3 particles avoided the sedimentation and agglomeration of the fillers through electrostatic and steric forces [32]. The acidic IL had a better effect on the particle dispersion than the basic IL.
Amino acid ionic liquids (AAILs) were adopted as the core to modify nanoparticles of polymers of intrinsic microporosity (AAILs@PIM (core-shell) CNPs). The AAILs provided high CO2 adsorption selectivity, while the PIM shell avoided its loss [93].
In some cases, a dual modification was concurrently performed, as in the work on Pebax/PEG 400/NH
2 separation. These researches were reviewed in 2018 by Kardani et al. [2] and in 2022 by Bernardo and Clarizia.
-MIL125 nanocomposite membranes in which polyethylene glycol was blended with Pebax, while the filler was amino functionalized [73].
Another approach to solve the problem of nanoparticle agglomeration is the synthesis of hybrid materials such as liquid-like nanoparticle organic hybrid materials (NOHMs) with a polyetheramine canopy [16]. At the same time, Nanodiamonds modified with polyethyleneimine (PEI) had no visible agglomeration, as evidenced by SEM observation even at the highest loading of ND-PEI (1.5 wt%) [40]. The PEI layer on the ND surface successfully improved the interfacial adhesion and dispersion of the NDs in the Pebax matrix [40]. GO flakes functionalized with iron oxide (Fe3O4–GO) showed reduced interface defects due to a better interaction between the magnetically aligned GO composites and the Pebax matrix [55].
Pebax as host matrix takes advantages of a combined flexibility and mechanical resistance for the presence of Polyether and Polyamide units: the prevalence of one feature on the other depends on the relative amount of them. In addition, these polymers present intrinsic gas transport properties of interest for the separation of gaseous mixture containing CO
3. Operation Conditions
Operation conditions (e.g., temperature, pressure) influence the gas transport performance in both single and mixed gases.
Typically, as the temperature increases, the gas permeation rate rises as well, whereas the selectivity trend is the opposite. In fact, the diffusion term increases with temperature for all gas molecules, especially for the less permeable ones, as proven by the activation energy for the permeation process. On the other hand, the solubility term, linked to the condensability of the species, tends to decrease with the temperature, depressing the favorable contribution gained through the most adsorbable species.
MMMs based on ZnCo2O4 are more sensitive to temperature changes than the pure polymer [28].
A sharp increase in CO2 permeability was measured in MMMs based on amino acid ionic liquids encapsulated in a PIM shell, as evidenced by the increase in temperature in the range of 55–85 °C [93]. This was a consequence of a viscosity decay for the CO2–AAIL complex induced by the breaking of hydrogen bonds that chemically link CO2 molecules to the primary amines of the AAIL. Thus, if in the neat Pebax 2533, the increase in permeability is combined with a decrease in CO2/N2 selectivity, in the MMMs, it is associated with an increase in CO2/N2 selectivity, because nitrogen does not experience any further advantage in terms of the reduction in the viscosity with respect to a simple Arrhenius trend [93].
The incorporation of zeolite DD3R into the polymer lowers the activation energy for the permeation of CO2, which means that the transport in MMMs is facilitated. On the other hand, a much greater barrier (higher activation energy) to CH4 permeation was calculated by adding the zeolite particles [60].
The dependence from the operation pressure is not univocal: a pressure increase causes a denser polymer matrix with a reduction in both gas permeance and selectivity, as found for MMMs incorporating ZnCo2O4 [28] and for Pebax MMMs with optimal loading of [Hmim][NTf2]@LDH (6 wt%) [86]. Nevertheless, the MMM displays a smaller reduction than the neat Pebax membrane [86]. MMMs incorporating PEG and NaY showed a decline for the CO2 permeability with the increase in the trans-membrane pressure from 0.10 MPa to 0.25 MPa [58].
In Facilitated Transport membranes, a carrier saturation effect occurs at a high feed pressure, contributing to the deterioration of the CO2 separation performance [86,87].
In some cases, CO2 molecules can exert a plasticizing action on the polymer matrix when the pressure is raised. Accordingly, the separation selectivity declines, as evidenced in MMMs loaded with AA-LDH fillers for the CO2/CH4 separation [87].
An opposite behavior was observed for NH2-ZIF-8 dispersed at 10 wt% in Pebax 1657, showing an increase in CO2 permeability combined with an increase in selectivity moving from 1 to 9 bar [66].
An increase in the permeability of CO2 as a polar gas with the increasing of the pressure was observed in MMMs filled with GO-PPy due to the increase in gas sorption [54]. Instead, the permeability of the bulkier CH4 molecules was decreased due to the membranes having a more compact structure.
MMMs containing DD3R zeolites displayed a lower relative increase in CO2 permeability with the feed pressure than that of the neat Pebax 1074 [60]. This can be ascribed to the decrease in polymer-chain movement caused by the incorporation of zeolite particles. As a further consequence, a suppression of plasticization and swelling of the polymer matrix by CO2 was observed following the addition of the zeolite particles.
A positive effect on the permeability of both CO2 and CH4 was reported in the range of 4–10 bar for NH2-HNTs based membranes [90]. However, this increase was larger for CO2, thus leading to an increase in the ideal CO 2 [3].
Therefore, several promising fillers, capable of enhancing the structural and gas transport properties of the polymers establishing specific interactions with gas molecules, can be favorably incorporated within the host matrix. They include inorganic particles[4], carbon materials [5][6][7][8][9][10], zeolites[11][12]. An emerging trend is represented by 2D nanoparticles [13][14][15]. Other additives were recently explored to improve the separation performance of the Pebax matrix[16][17].
2. Fillers
An overview of the main filler types incorporated in Pebax polymers is reported in Figure 1. Particle type, size, shape and loading are the main factors that influence the MMM performance.
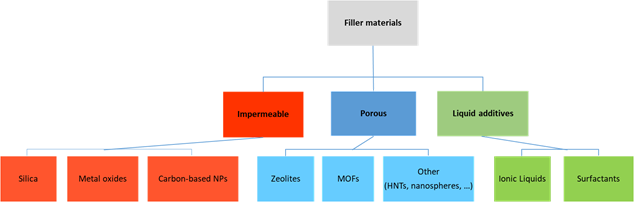
Figure 1. Filler typology used to prepare MMMs based on Pebax polymers[18].
Both porous and non-porous particles can be used in Pebax matrix, whereas the first ones offer additional routes to the permeating gas molecules, according to the opening size of their channels. On the other hand, the blocking of the pores must be absolutely avoided to maintain their greater effectiveness. Also surface and sorption properties suffer from this drawback.
In the field of porous fillers, for those having a high aspect ratio, their orientation plays a fundamental role. Significantly different transport rates are observed depending on whether the particles are arranged parallel or perpendicular to the membrane surface[19].
Generally, the presence of fillers imparts greater mechanical strength to the polymeric film, reducing its flexibility.
An increasing loading, typically, produces a progressive permeation rate/performance enhancement, before achieving agglomeration or sticking phenomena, at high filler content, that are detrimental for selectivity target. The maximum loading depends on the filler type.
At the same size and content, synergistic effects are observed in the case of filler combinations versus single fillers in terms of better sorption properties and creation of selective gaps for CO
/CH4 selectivity due to the concomitant effect of a larger CO
2[20].
Filler pretreatment may be necessary in order to improve the dispersion within the polymer matrix, but it is not as stringent as in the case of glassy polymers. However, alternatively, appropriate physical linkages or specific chemical bonds facilitate the compatibility between the heterogeneous phases, improving the dispersion of the individual fillers and preventing particle agglomeration, but also modifying favorably the original polymer crystallinity or the free volume available for the gas molecules, with advantages in terms of final separation performance. Another aim of the filler functionalization is the introduction of chemical moieties for promoting the selective transport of CO
sorption capacity for the more condensable species.
Composite MMMs based on MOFs in Pebax 1657, tested for CO
2 with respect to other gases.
Specific additives, such as ionic liquids or PEGs, increase the mutual compatibility between the phases [21][22]. At the same time, they can make the matrix more flexible and convey the transport of some species when they bring appropriate functionalites[23].
Operation conditions affect the performance of the MMMs in a multiple way.
In a polymer matrix, as temperature increases, gas permeability rises, particularly for less permeable molecules. Indeed, gas diffusion is an activated process favored by temperature and it represent the main factor in which the permeability can be decoupled. The other term, the solubility, is negatively affected by the temperature, that reduces the sorption capacity of the species in the polymer matrix. As result of this combined effect, a decay in selectivity was observed.
The presence of the fillers can modify the behavior of the original matrix versus the gas permeation, also depending on additional transport mechanism occurrence. Therefore, unusual increases in CO
/CH4 separation at different feed pressures (3–5 bar), showed an improvement in both the CO
2/N
2 selectivity were observed at high temperature (e.g., 65°C[24]).
For what concerns an increase of the feed pressure, it causes a “densification” of the host matrix with a reduction of the permeation rate[13]. Similar trend was observed when carrier saturation occurs, combined to a separation factor decay [25][26]. Nevertheless, in the case of functionalized fillers, opposite behaviors can happen as effect of enhancement of gas sorption properties of specific polar gases (e.g. carbon dioxide) with respect to non-polar species (e.g. nitrogen or methane)[27][28].
Wet-state separations show a significantly different separation extent in comparison with the same operation carried out in dry-state. The humidity tends to swell the polymer causing lower resistance to the passage of gas molecules[29].
Commonly, the plasticization and swelling phenomena, occurring when CO
/CH4 selectivity with increasing pressure due to the enhanced CO
2 permeates through the polymer matrix at moderate temperature and pressure conditions, are reduced as result of nanoparticles presence in MMMs, expanding their field of application.