The last decade has seen rapid developments in the areas of carbon fiber technology, additive manufacturing technology, sensor engineering, i.e., wearables, and new structural reinforcement techniques. These developments, although from different areas, have collectively paved way for concrete structures with non-corrosive reinforcement and in-built sensors. This effort bridges the gap between civil engineering and sensor engineering communities through an overview on the up-to-date technological advances in both sectors, with a special focus on textile reinforced concrete embedded with Fiber Optic Sensors (FOSs). These have advantages over alternative concepts, such as, immunity to electromagnetic interference or light weight. The former is an important aspect for some civil infrastructure, i.e., railways where the lines are electrified. Thus, there has been in depth research on the feasibility and deployment of FOSs for SHM purposes. The characteristics desired for the ideal FOS for strain measurement in civil structures would include: adequate sensitivity and dynamic range; linear response; sensitivity to the direction of measurand field change; being single-ended, i.e., to minimize the number of leads; insensitivity to thermal fluctuations; non-perturbativity to the structure; immunity to power interruptions; ability to multiplex; ease of mass production, and durability for the entire lifetime of the structure.
1. Introduction
The development of optical fiber saw a rapid increase in the 1990s for telecommunications purposes. Indeed, most of the optical sources, detectors, and related consumables that are still in use, i.e., connectors, couplers, etc., operate at the wavelengths that were initially focused on telecommunication windows, i.e., 1550 nm and 1300 nm. In recent years, fiber optics has also drawn considerable attention in the sensors field owing to its many advantages over the conventional electrical-based sensors, such as immunity to electromagnetic interference, multiplexing capability, high temperature capability, chemical inertness, robustness, and light weight. A FOS system consists of a transducer device, i.e., the sensing element or mechanism, a communication channel which carries the measurand data, and a subsystem that provides energy and detects/processes and conditions the received signal. Since several sensors can be embedded along a single fiber, creating a distributed sensors network, the detection of local damage such as strain, cracks, and corrosion, becomes a possibility. The conventional electrical sensors must have two wires for each sensor to form an electrical loop. In comparison, the multiplexing capability of the optical fiber network is far easier to implement and install. FOSs can be used either as point sensors or in a distributed sensor configuration, making the design of the sensor or the sensor network more flexible and application specific. Thus, depending on the transduction mechanisms used, a variety of physical and chemical sensors can be developed, which have potential applications in a wide range of industries.
The use of FOSs for the SHM of concrete was first suggested by Mendez et al. (1990)
[1]. Since the first development from Mendez et al., many different FOSs have been conceived for SHM of concrete through the measurement of cracks
[2], strains
[3][4] as well as Relative Humidity (RH)
[5] and pH
[6][7], for instance. In general, the FOS concepts can be categorized into (i) integrated, (ii) point, (iii) quasi-distributed and (iv) distributed sensing, depending on the spatial distribution and sensing mechanism
[8]. The different categories of FOSs and their corresponding sensing mechanisms are summarized in
Figure 61, followed by an explanation on their operating mechanisms and their utilizations in exemplary applications.
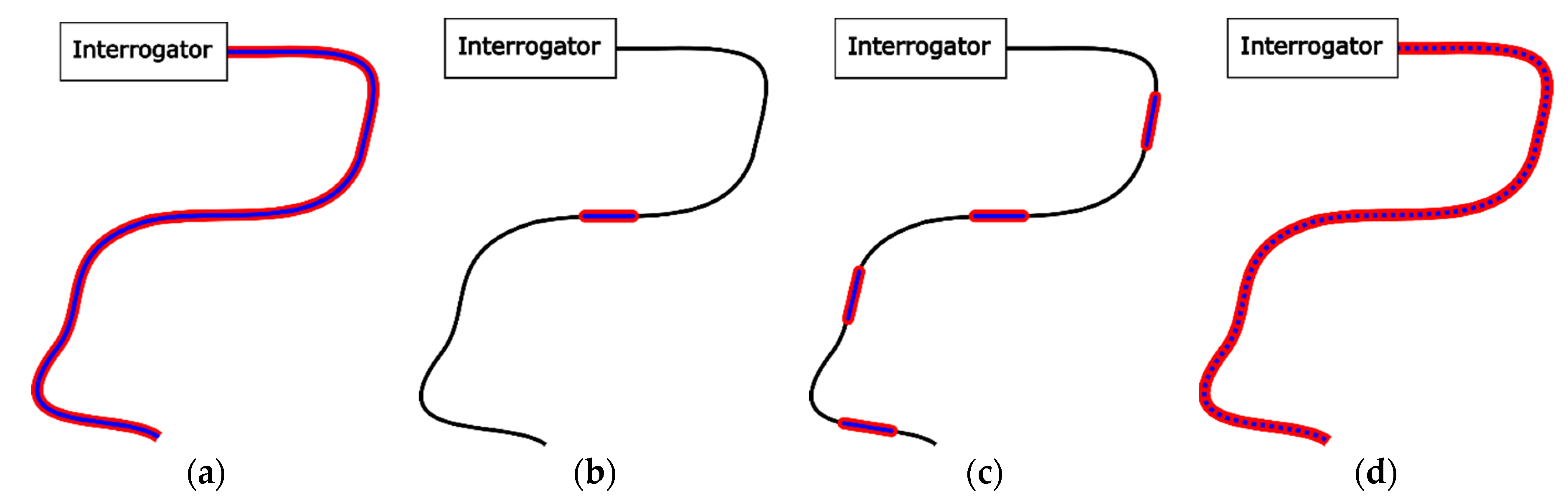
Figure 61. Graphical illustration of different FOS concepts: integrated (a), point (b), quasi-distributed (c), and distributed (d). The black line illustrates the fiber optic link. The red and blue lines represent the sensing length and measurement points respectively.
2. Fiber Optic Sensors Embedded in Textile-Reinforced Concrete for Smart Structural Health Monitoring
The recent growth in the wearable sensor technology market, especially in the field of biomedical and robotics sectors, indicates a clear direction that could be predicted for civil infrastructure of the future. It can be inferred that textile reinforcement will take over its conventional counterparts, and civil infrastructure will be fully embedded with sensors and other capabilities to ensure its safety, comfort, and long-term durability while minimizing the otherwise expected carbon footprint. In order to achieve these targets, reliable and cost-effective sensors need to be in place. Much like the concrete reinforcement sector, the infrastructure monitoring sensors sector has also seen a dramatic shift towards new methods of sensing, i.e., adopting fiber optic sensors as opposed to conventional SHM schemes such as strain gauges.
In terms of functionalizing these reinforcement structures with sensors for SHM applications, FOSs are the best candidates due to their advantages, such as their small size, light weight, and remote interrogation and multiplexing capability. Optical fibers can be integrated during the manufacturing process of the TRCs in large volumes or could be tailor-made. Thus, such structures can be fabricated economically and optimized for specific target applications. When FCSs are equipped with optical fibers, Bremer et al. demonstrated in
[9] that the corresponding sensor response depends linearly on the applied strain with a relatively low hysteresis and, depending on the integration direction, the load direction can be discriminated between transverse and longitudinal loads. Moreover, in terms of distributed sensing, it could also be verified that integrated optical fibers can be applied to determine the applied strain profile. However, depending on the magnitude of the applied load, the obtained load peak tends to broaden, and this reduces the spatial resolution. Therefore, in
[10] it was deduced that for the FOS response, the bonding length between the optical fiber and the textile-base reinforcement structure is important, i.e., that the bonding length is long enough and thus avoids directional variations of the optical fiber at the point of measurement, so that the applied load can be sufficiently transferred on to the FOS. Moreover, it was demonstrated that when embedding the functionalized textile-based carbon reinforcement structure into highly alkaline concrete, the applied PVA coating, which is relatively inert, provides substantial resistance against deterioration of the structure
[10]. In addition, functionalized TNS designed for the reinforcement and crack detection of concrete structures have been reported in
[2]. The optical glass fiber was pre-strained during the fabrication process of the functionalized TNS in order to enhance the crack detection mechanism. Furthermore, when embedding the functionalized TNS into concrete, an insignificant light attenuation of 0.09 dB and a relatively high sensitivity of crack detection, i.e., of ≥1.4 mm, was observed. The performance of functionalized TNS and FCS are summarized for comparison in
Table 1. Future directions of functionalized TRCs clearly point towards increased exploitation of Rayleigh, Brillouin, and/or Raman scattering for fiber-optic sensor networks, their implementation for structural health monitoring, as well as the combination with artificial intelligence algorithms for signal analysis and sensitivity enhancement, see e.g.,
[11][12]. In this context, several aspects are worth to be highlighted as they offer the potential to significantly enhance the performance of sensors based on laser-optical principles in comparison to state of the art technology. Firstly, the unique properties of laser radiation, as demonstrated already in many precision measurement applications from very diverse research fields, e.g.
[13][14][15][16][17][18][19], are not fully exploited in civil infrastructure monitoring or embedded sensing and are expected to lead to a noticeable increase in measurement accuracy in the latter areas as well, in particular, in view of the continued rapid development in laser technology, e.g.
[14]. Secondly, the trend towards miniaturized multiplexed sensing might open a route to the simultaneous determination of a multitude of physical or chemical quantities, e.g. strain, pressure, temperature, refractive index, humidity or pH value, with high spatial and temporal resolution
[20][21][22][23]. Thirdly, the combination of integrated photonic concepts with ubiquitous read-out units [e.g.
[24][25] and/or artificial intelligence, i.e. from neuromorphic computing
[26], could allow for real-time dynamic monitoring of large infrastructure in possibly harsh environments and ultimately lead to application-specific fiber-optic sensor networks for precision monitoring, surveillance or remote sensing as well as efficient predictive data analysis, see e.g.
[11][12][27][28] and references therein. Also, these developments may help to reduce the resource and energy expenditure for large-scale distributed sensing architecture in versatile application scenarios which, in the end, could lead to transfer of such measurement systems to the mass market at affordable cost and equipment requirements.
Table 1. Comparison of functionalized TNS and FCS. Values obtained from
[2][29][30][10].
Host Structure |
Textile Net Structures (TNS) |
Functionalized Carbon Structure (FCS) |
Sensing mechanism |
Point-based (e.g., power meter) andDistributed (OTDR) |
Point-based (e.g., FBG, EFPI),Quasi-distributed (FBG network), andDistributed (e.g., DAS, OFDR, BOTDA) |
Sensing parameter |
Cracks |
Strain |
Sensitivity |
≥1.4 mm |
0.44 nm/% |
Hysteresis |
Not specified |
0.011% |
Spatial resolution |
Not specified |
≤0.5 m for distributed sensing |
Fiber type |
Single-mode optical glass fiber |
Single-mode optical glass fiber |
Optical attenuation |
≤0.09 dB |
Insignificant light attenuation due to connector tolerances |
Fabrication technology |
Reel-to-reel knitting technique |
Modified embroidery technique |
Estimated cost |
Low |
Moderate |
Highlight |
Pre-strained optical glass fiber |
Relatively inert due to PVA coating |