1. Introduction
Coronaviruses (CoV) are a large family of viruses that may cause disease in animals or humans
[1][2][3][1,2,3]. They can provoke respiratory infections ranging from the common cold to more severe illnesses
[3]. The novel coronavirus, called SARS-CoV-2, which emerged in December 2019 causing coronavirus disease 2019 (COVID-19), can lead to serious, even fatal, disease
[4][5][6][4,5,6], and was declared a global pandemic by the World Health Organization on 11 March 2020.
All coronaviruses possess an enveloped, positive-sense, single-stranded RNA genome encoding for 4 structural membrane proteins, i.e., Spike (S), envelope (E), membrane (M) and nucleocapsid (N) proteins
[7]. The Spike proteins S are essential for viral entry into host cells, which occurs essentially through binding to the angiotensin-converting enzyme ACE2
[8][9][10][11][8,9,10,11]. ACE2 is present on the surface of multiple cell types, including respiratory and intestinal epithelial cells, endothelial cells, kidney cells (renal tubules), cerebral neurons, and immune cells, such as alveolar monocytes/macrophages
[12][13][12,13].
Therefore, bioactive compounds able to inhibit the interaction between the COVID-19 S protein and the ACE2 receptor may be precious drugs for effective antiviral therapeutic strategies
[14]. Indeed, human neutralizing antibodies targeting S protein and blocking SARS-CoV-2 cellular entry are promising therapeutic tools
[15][16][17][18][19][15,16,17,18,19].
After attachment of the virus, a proteolytic enzyme of the host cell, mainly type II transmembrane serine protease TMPRSS2, cleaves and activates the receptor-attached Spike macromolecule
[20]. This protease, anchored in the cell membrane near ACE2 receptors, and expressed in the epithelial cell lining of the nose, trachea and distal airways, cleaves SARS-CoV-2 S protein into two subunits, S1 and S2, respectively. The N-terminus of S1 subunit represents the receptor-binding domain (RBD) which binds to ACE2, whereas S2 subunit serves to promote fusion activity via its
C-terminus
[20].
Drugs able to bind key regions of the selected targets with high affinity and specificity could in principle sterically block the binding sites of the viral/host proteins or induce conformational switches in the biomolecules avoiding their correct recognition. Various works have already investigated, experimentally or in silico, the effects of natural compounds or synthetic drugs on COVID-19-related targets
[21][22][23][24][25][21,22,23,24,25]. Several natural products endowed with significant biological activities, especially extracted from plants, have been thus identified as potentially able to contrast the dissemination of Coronavirus and, at the same time, enhance immunity, stimulating further screenings to discover new candidate drugs.
Natural polyphenols are an abundant and widely distributed family of bioactive molecules, whose structure is generally constituted by one or more aromatic rings carrying one or more hydroxyl groups
[26]. Two natural stilbene polyphenols that have attracted much attention, especially for their manifold biological properties, are trans-resveratrol (here named RESV, 3,5,4′-trihydroxystilbene)
[27] and trans-polydatin (here named PD, 3,5,4′-trihydroxystilbene-3-β-
D-glucoside,
Figure 1)
[28]. These polyphenols were originally isolated from the root and rhizome of Polygonum cuspidatum, a plant used in traditional Chinese medicine for its analgesic, antipyretic and diuretic properties. Resveratrol is a phytoalexin produced by more than 70 plants in response to various stresses and is found in a variety of foods, including red grapes, peanuts, pistachios, red wine, blueberries, cranberries, and even cocoa and dark chocolate
[29]. Polydatin is a glycosylated form of RESV and the most abundant derivative of resveratrol in nature
[30].
Figure 1. Chemical structures of (a) trans-resveratrol (RESV) and (b) trans-polydatin (PD).
Many studies have been carried out on the beneficial effects of these polyphenols on the human body (e.g., anti-oxidant, anti-inflammatory, antitumor, antiviral, neuroprotective, hepatoprotective and ischemia preventing activities), and on their mechanisms of action
[27][28][31][32][33][27,28,31,32,33].
Analogously to other polyphenols, RESV has limited bioavailability and poor water solubility
[34]. On the other hand, PD displays higher water solubility and metabolic stability, as well as better oral absorption than RESV and is used in clinics with no side effects
[35][36][35,36].
These compounds were recently proposed as potential drugs against COVID-19-related targets as indicated by preliminary in silico studies and cellular assays
[37][38][39][37,38,39]. Furthermore, polydatin and resveratrol treatments could be beneficial for COVID-19 infection also due to their anti-inflammatory activities particularly in the respiratory tract
[40][41][42][43][44][45][46][47][48][49][40,41,42,43,44,45,46,47,48,49].
On these bases, we here investigated—by means of detailed in silico studies and preliminary biochemical assays—the potential of RESV and PD to bind ACE2 and/or Spike proteins interfering with their interaction, essential for virus host-cell entry. To the best of our knowledge, this is the first report exploring, with preliminary experimental assays, the interference of PD/RESV on the binding of a COVID-19 key protein to a host target.
In particular, we here studied the interactions of PD and RESV with both Spike and ACE2 as separated proteins as well as with their complex through a molecular docking-based computational approach, using the available molecular structures as deposited in the PDB database. Furthermore, preliminary biochemical assays, i.e., ELISA-like assays employing the target recombinant proteins (Spike S1 subunit and ACE2) and the tested small-molecules, were performed to evaluate the ability of PD/RESV to inhibit/block the ACE2 recognition by Spike.
2. Molecular docking simulation.
Spike-protein pre-fusion conformation [50,76] is a trimer constituted of two subunits, S1 and S2, which are cleaved following receptor binding [77]. S1 Receptor Binding Domains (RBDs) host the binding motifs (RBMs) able to recognize ACE2. The high RBD flexibility allows the Spike to sample open or closed conformations, in which RBMs are respectively exposed or hidden inside the protomers interface [77–81].
Therefore, the binding to SARS-CoV-2 Spike structure with one RBD in an open conformation (PDB ID: 6VSB [50]) has been investigated by molecular docking simulations. The pockets on the RBD surface appear able to accommodate both PD and RESV ligands (Figure 2). A slightly lower affinity for the RBD domain was found for RESV (−6.5 kcal/mol for the best docking pose, Table 1) with respect to PD (top-ranked pose −6.9 kcal/mol).
Table 1. Docking scores (i.e., the approximate binding energy estimated by the docking scoring function, kcal/mol units) for PD and RESV top-ranked docked poses.
|
PD
|
RESV
|
Spike RBD
|
−6.9
|
−6.5
|
ACE2
|
−8.4
|
−6.9
|
S:ACE2 region I
|
−8.1
|
−7.6
|
S:ACE2 region II
|
−6.9
|
−6.5
|
ACE2 homodimer (PDB ID: 6M18, [51]) is constituted by an N-terminal protease domain, involved in the interaction with SARS-CoV-2 Spike RBD [51], and a C-terminal collectrin-like domain, comprising the ACE2 transmembrane helix. Molecular docking simulations were performed near ACE2 protease domain α1 and α2 helices, thus far from the catalytic site related to ACE2 physiological function. Despite its size, PD can be easily accommodated in a deep groove behind the two helices (Figure 3a,b) producing a high docking score (−8.4 kcal/mol, Table 1). RESV can also bind to the pockets near α1 and α2 helices, but with a ~1 kcal/mol lower score with respect to PD (−6.9 kcal/mol, Table 1) (Figure 3c,d).
Figure 3. PD (a,b) and RESV (c,d) top-ranked poses docked to ACE2.
Both RESV and PD showed a good estimated binding affinity in the groove between the Spike RBD and ACE2. Due to the high spatial extent of the Spike:ACE2 interface, docking simulations were performed in two distinct regions (named regions I and II). In the first interfacial region investigated (region I), the most stable RESV binding mode involves recognition of one of the interfacial pockets through polar and hydrophobic contacts (Figure 4c,d). PD binds in the same pocket as RESV (Figure 4a,b) but interacting with more surrounding residues, thus showing a higher docking score (−8.1 kcal/mol, Table 1), compared to that of RESV (−7.6 kcal/mol).
Table 1. Docking scores (i.e., the approximate binding energy estimated by the docking scoring function, kcal/mol units) for PD and RESV top-ranked docked poses.
| PD | RESV |
---|
Spike RBD | −6.9 | −6.5 |
Spike A/B interface | −7.3 | >−6.5 |
Spike A/C interface | −7.3 | >−6.5 |
ACE2 | −8.4 | −6.9 |
S:ACE2 region I | −8.1 | −7.6 |
S:ACE2 region II | −6.9 | −6.5 |
Figure 4. PD (a,b) and RESV (c,d) top-ranked poses docked to Spike:ACE2 interface region I.
In the second region (region II) investigated at the interface between viral Spike RBD and human ACE2, the top-ranked RESV conformer fits into a large ACE2 cavity far from the interaction sites between the two protein domains. On the contrary, in the best PD binding mode, the ligand binds onto a pocket located at one end of the ACE2:Spike complex interface with a docking score 0.4 kcal/mol lower than RESV (−6.9 kcal/mol, Table 1). It is interesting to note the fundamental role played by the glucosidic moiety which interacts with the residues of the main chains that are involved in the formation of the ACE2:Spike dimer. Weakening of these interactions could, in principle, contribute to the dissociation of the dimeric complex.
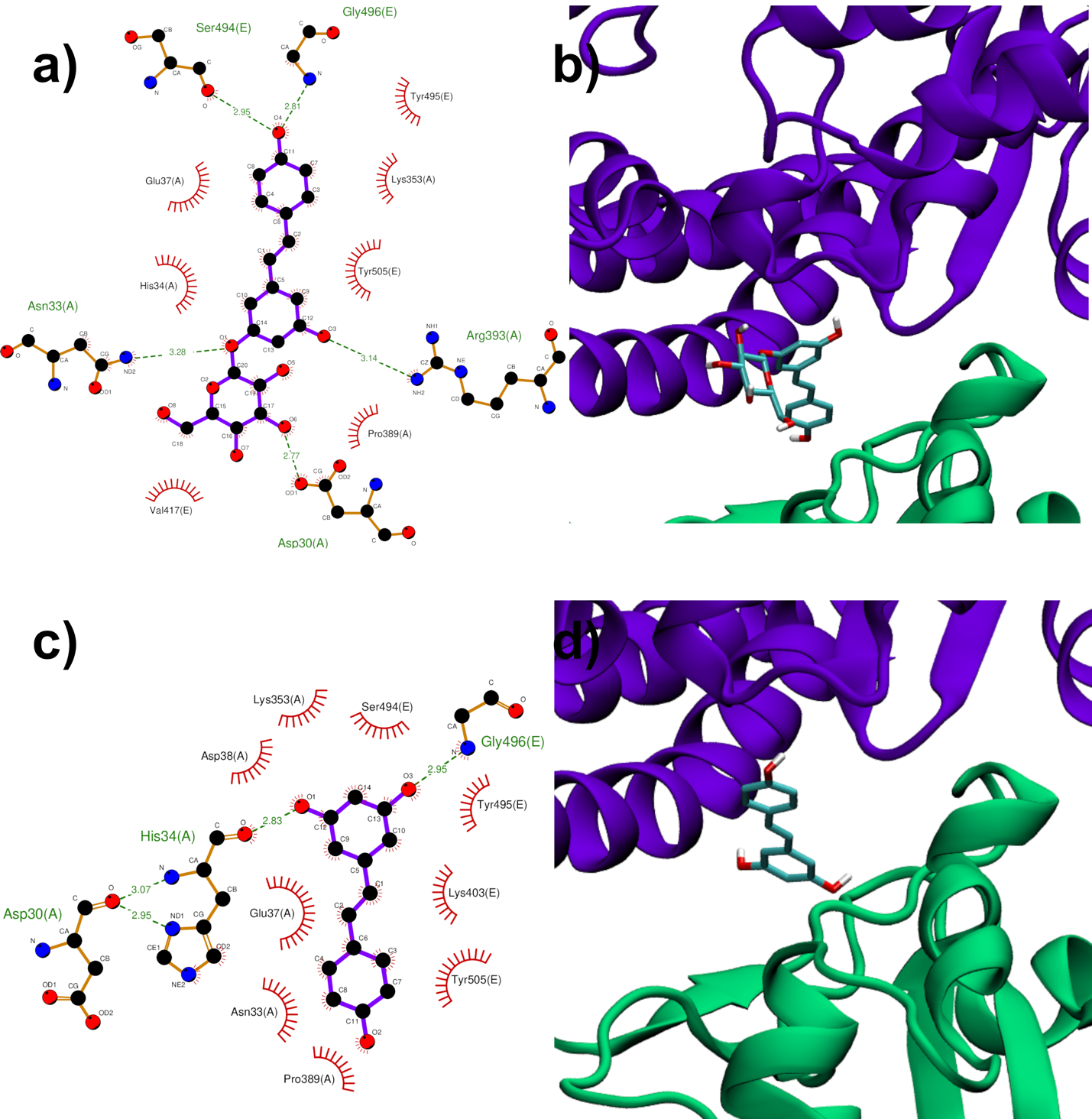
Figure 4. PD (a,b) and RESV (c,d) top-ranked poses docked to Spike:ACE2 interface region I. Two-dimensional interaction maps (a–c): C, N and O atoms are reported in black, blue and red, respectivelts from docky. Hydrogen bonds are depicted as green dashed lines, while hydrophobic interactions as red cogwheels. Hydrogen atoms are not depicted for ease of illustration. The names of proteins’ residues involved in interactions with the ligand are reported. Three-dimensional representations of PD and RESV docked poses (b–d): proteins’ backbone is represented as a cartoon, while the lig siand as sticks.
Results from docking simulations on the already assembled Spike:ACE2 complex reveal the potential capability of PD, but also RESV, to insert themselves into the extended adduct interface. This leads to the hypothesis of a ligand-induced dissociation or weakening effect, through allosteric (such as for region I) or direct (region II) interference.