1. Microorganism-Mediated Effect on Productive Sectors
Climate variations (e.g., temperature increase, rainfall instability, glacial retreat, extreme weather events, etc.) have foreseeable impacts on certain industries. These impacts encompass technical concerns (e.g., power supply) or extend to more severe problems in sectors such as agriculture, forestry, livestock and commercial fishing, which are primary sectors of great economic relevance, whose global value grew by 73% between 2000 and 2019, reaching USD 3.5 trillion in 2018 [1]. Nowadays, farmers and scientists are already both turning their eyes to the use of biofertilizers and eco-friendly tillage practices in order to protect soil health, reduce GHG emissions and increase carbon sequestration [2]. However, the increase in outbreaks of several diseases, as well as the geographical expansion of different pathogens, is compromising the balance between eco-friendly activities and the way to face these new climate-change‑derived risks [3] (Figure 1). Thus, the microorganisms involved in these processes play a crucial role as detailed below.
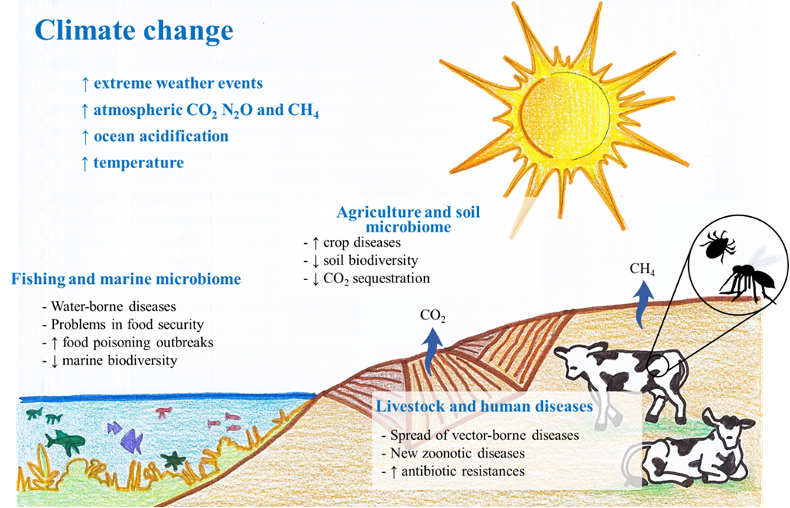
Figure 1. Main effects of climate change on microorganisms: (i) expansion and increase in waterborne diseases affecting fish and shellfish and causing an increase in food poisoning outbreaks and loss of marine biodiversity; (ii) expansion and intensification of pathogenic fungal infections in crops all around the world; and (iii) outbreaks of livestock diseases, emergence of new zoonotic diseases and increase in antibiotic resistance.
2. Agriculture and Soil Microbiome: Eternal Feedback
One of the most important worldwide primary sectors is agriculture, which relies on food production. However, the variation in soil microbial communities influenced by climatic change affects the physiology, temperature sensitivity or plant growth rate, which jeopardizes the final yields and compromises the future of this sector [4]. Thus, increased temperatures accelerate microbial decomposition activities, leading to faster CO2 emissions. As a result, soils will become a carbon dioxide source rather than a sink [4]. This CO2 enrichment enhances the development of rhizobial populations and the increase in N-fixing microorganisms in controlled environments, although multiple resource limitations dampen rhizobial responses in natural systems. In contrast, high temperatures lead to drought, which reduces colonization by arbuscular mycorrhizal fungi [4][5].
In addition, temperature and soil humidity play a critical role in microbial-soil abundance, diversity and metabolic functionality, since climatic factors greatly modify the type and quantity of some plant species that predominate in a landscape [6][7]. Furthermore, the consequences of climate change on soil communities and nutritional balances depend on the specific soil type, vegetation cover and management techniques. As a result, the total effects of climate change on soil communities and nutritional balances seem logical but unclear [4][5][8].
The assessment of climate change's impact on crop yields involves the examination of various models. Despite some inconsistencies in their final projections, these models forecast substantial financial losses in numerous crops worldwide due to the migration of pests and pathogens to new geographical regions
[9][10][11][12][13][14]. However, while there are challenges in precisely quantifying the potential consequences of climate change on plant pests, crop production, and threats to natural biodiversity
[15][16], several bacterial and fungal diseases have already initiated their geographic expansion. It's worth noting that most of these expansions cannot yet be directly attributed to climate change (
Table 1).
Table 1.
Geographical expansion of agricultural diseases reported in recent years.
Disease
|
Pathogen
|
Host
|
Origin
|
Spread and Development
|
Ref.
|
Ash dieback
|
Hymenoscyphus fraxineus
|
Ash trees
|
Asia
|
Asia, Europe and Africa
|
[22][23]
|
Bacterial blight or Bacterial leaf blight
|
Xanthomonas oryzae
|
Rice
|
Japan
|
Worldwide (especially Asia and Africa)
|
[24][25]
|
Bacterial canker
|
Pseudomonas syringae
|
Fruit trees
|
Depends on pathovar
|
Worldwide
|
[26][27]
|
Brown rust or Leaf rust
|
Puccinia recondita
|
Cereals (wheat, rye and barley)
|
Eastern Australia
|
Worldwide
|
[28][29]
|
Brown spot
|
Bipolaris oryzae
|
Rice
|
USA
|
Asia, Europe and South America
|
[17][30]
|
Bunch rot or Gray mold
|
Botrytis cinerea
|
Wide range
|
Unknown
|
Worldwide
|
[31]
|
Chestnut canker
|
Cryphonectria parasitic
|
Chestnut tree
|
Asia
|
North America
|
[32]
|
Disease dependent on the
Diaporthe species
|
Diaporthe spp.
|
Wide range
|
Germany
|
Europe, Australia and Asia
|
[20][33]
|
Disease dependent on the X. fastidious subspecies
|
Xylella fastidiosa
|
Wide range
|
USA
|
South and North America and Europe
|
[20][34]
|
Downy mildew
|
Plasmopara viticola
|
Grape
|
North America
|
Worldwide
|
[35][36][37]
|
Dry root rot
|
Rhizoctonia bataticola (also Macrophomina phaseolina)
|
Chickpea
|
India
|
North America, Asia and Africa
|
[38]
|
Dutch Elm disease
|
Ceratocystis ulmi (also Ophistoma ulmi)
|
Elm
|
Asia
|
Worldwide
|
[39]
|
Fire blight
|
Erwinia amylovora
|
Apple, Pearl and some Rosaceae
|
North America
|
Europe and Asia
|
[40]
|
Rice blast
|
Magnaporthe oryzae (anamorph Pyricularia oryzae)
|
Rice
|
Brazil
|
South America, Asia, Africa and Europe
|
[20][41]
|
Stewart’s wilt
|
Pantoea stewartii (formerly Erwinia stewartii)
|
Corn
|
USA
|
Italy, Malaysia
|
[42][43]
|
Yellow rust or Stripe rust
|
Puccinia striiformis
|
Wheat
|
Transcaucasia (Armenia, Georgia and Azerbaijan)
|
Worldwide
|
[28][44]
|
Wheat blast
|
Pyricularia graminis-tritici
|
Wheat
|
Brazil
|
North and South America and Asia
|
[45]
|
Fungi annually destroy one-third of all food crops, including rice, wheat, maize, potatoes and soybean. The mitigation of this loss would have been enough to feed around 8.5% of the world population of seven billion in 2011
[14]. Some models create a devastating scenario for 2050, with a large increase in fungal infections throughout Europe, such as the following:
-
Brown rust (mainly caused by Puccinia recondita), in the case of wheat, is forecast to increase its pressure on the crop by 20–100%, and yellow rust (caused by Puccinia striiformis) will increase by 5–20% in cold regions.
An increase in cases of diseases in leafy vegetable and cereal crops has already been reported in Italy, as a result of several pathogens’ effect, such as
Plectosphaerella cucumerina,
Alternaria sp.,
Fusarium equiseti, Myrothecium verrucaria, Myrothecium roridum, Phoma valerianellae, Pleospora betae, Peronospora belbahrii and
Pythium ultimum, as well as the appearance of new pathogens like different species of
Pythium (
Pythium aphanidermatum, Pythium irregulare, Pythium dissotocum, Pythium coloratum, Pythium diclinum or
Pythium lutarium) and new species causing yellow rust or stem rust
[17][
Rice pathogens such as Pyricularia oryzae (the main cause of blast) and Bipolaris oryzae (or brown spot) are favored in all European rice districts, with the most critical situation in northern Italy (with an increase of close to 100%).
-
-
Among the new infections that have been reported in recent years in southern Europe and the Mediterranean coast, different species of the fungus
Diaporthe have been found infecting several citruses, and the bacterium
Xylella fastidiosa has also been discovered in olive trees
[20].
In the case of grape,
Plasmopara viticola (also called downy mildew) will increase by 5–20% across Europe, while
Botrytis cinerea (or bunch rot) will have diverse impacts, ranging from a 20% decrease to a 100% increase in infection events
[17].
Indeed, the spread of several agricultural diseases toward northern regions of Europe has already been reported:
On the other hand, fungal infections of invertebrate hosts also require attention, not only because they are an important route of transmission of pathogens to new areas but also because they can boost agricultural crises due to ecological imbalance. Thus, a dramatic example is bee broods, which are susceptible to some fungal infections, like those caused by
Ascosphaera and
Aspergillus, and viruses, such as Deformed wing virus (DWV) or Varroa destructor virus-1 (VDV1). Thus, agricultural production is highly dependent on bee pollination, and infections may lead to unprecedented disasters (see below)
[6][15][21].
In summary, the causative agents of plant diseases are troublesome travelers that result in huge economic losses for the agricultural and forestry sectors, although obtaining realistic analyses seems complex.
3. Livestock and Climate Change: An Arthropoda Matter
The livestock sector is a capital pillar of the global food system, which, according to the FAO
[1], represents 40% of the global agricultural output value and supports the livelihood, food and nutrition security of almost 1.3 billion people
[1]. Although the increase in productivity efficiency helps to minimize the detrimental environmental impact of livestock, global farm-gate GHG emissions increased by 11% between 2000 and 2019, and around 55% of them are related to the livestock industry
[46][47].
As in the agricultural sector, climate change could directly and indirectly influence livestock production in many ways: (i) growth performance, (ii) the yield and quality of the product, (iii) reproductive performance and (iv) health status. However, the main risk of climate change, as far as microorganisms are concerned, is an increase in fungal and bacterial diseases
[46]. In addition, changes in temperature may trigger the secretion of stress hormones such as cortisol, which suppresses the immune system and favors pathogen infections, which also increases their transmission and expansion
[48].
Pathogens’ geographical extension is sometimes attributed to both anthropogenic and natural effects, including climatic factors and fauna and flora spread. Hence, the increased temperatures in the northern regions favor the expansion of numerous pathogen vectors (e.g., insects) promoting the geographic spread and increasing the incidence of some infections and diseases
[49] (
Table 2).
Table 2.
Geographical expansion of livestock diseases reported in recent years.
Disease
|
Pathogen
|
Vector
|
Host
|
Origin
|
Spread and Development
|
Ref.
|
Anaplasmosis
|
Anaplasma phagocytophilum
|
Ixodes scapularis, Ixodes pacificus
|
Sheep and cattle
|
Scotland
|
Worldwide
|
[50][51]
|
Babesiosis
|
Babesia microti, Babesia venatorum and Babesia divergens
|
Ixodes ricinus, I. scapularis
|
Mammals
|
Romania
|
Europe and North America
|
[52]
|
Babesia bovis and Babesia bigemina
|
Rhipicephalus microplus
|
Mammals
|
Asia, Africa, South and Central America
|
Europe and North America
|
[53]
|
Bluetongue Virus
|
Orbivirus
|
Culicoides imicola
|
Ruminants
|
South Africa
|
USA, Canada, Australia, South and Central Europe
|
[54]
|
Canine Babesiosis
|
Babesia spp.
|
Dermacentor reticulatus
|
Mammals (especially cattle) and birds
|
Romania
|
Worldwide
|
[55]
|
Colorado tick fever
|
Coltivirus
|
Dermacentor andersoni
|
Mammals
|
Western US
|
Europe and North America
|
[56][57]
|
Ehrlichiosis
|
Ehrlichia chaffeensis and Ehrlichia ewingi
|
Amblyomma americanum
|
Mammals
|
Canada
|
North America and Europe
|
[58][59]
|
Leptospirosis
|
Leptospira spp.
|
Environmental transmission
|
Mammals
|
Japan and Europe
|
Asia, Australia, America and South Europe
|
[60][61]
|
Lyme disease
|
Borrelia burgdorferi
|
Ixodes scapularis
|
Rodents
|
USA
|
North America and Eurasia
|
[62][63][64]
|
Powassan virus disease
|
Powassan virus
|
Several tick species
|
Mammals
|
Unknown
|
North America
|
[65]
|
Q fever
|
Coxiella burnetii
|
D. reticulatus
|
Ruminants (cattle, goat and sheep)
|
Australia
|
Europe and North America
|
[66]
|
Rocky Mountain spotted fever
|
Rickettsia rickettsii
|
D. andersoni
|
Mammals
|
South and Central America
|
North America
|
[67]
|
Rickettsia parkeri
|
A. maculatum
|
Central and North America
|
Disease
|
Pathogen
|
Vector
|
Host
|
Origin
|
Spread and Development
|
Ref.
|
Anaplasmosis
|
Anaplasma phagocytophilum
|
Ixodes scapularis, Ixodes pacificus
|
Sheep and cattle
|
Scotland
|
Worldwide
|
[50][51]
|
Babesiosis
|
Babesia microti, Babesia venatorum and Babesia divergens
|
Ixodes ricinus, I. scapularis
|
Mammals
|
Romania
|
Europe and North America
|
[52]
|
Babesia bovis and Babesia bigemina
|
Rhipicephalus microplus
|
Mammals
|
Asia, Africa, South and Central America
|
Europe and North America
|
[53]
|
Bluetongue Virus
|
Orbivirus
|
Culicoides imicola
|
Ruminants
|
South Africa
|
USA, Canada, Australia, South and Central Europe
|
[54]
|
Canine Babesiosis
|
Babesia spp.
|
Dermacentor reticulatus
|
Mammals (especially cattle) and birds
|
Romania
|
Worldwide
|
[55]
|
Colorado tick fever
|
Coltivirus
|
Dermacentor andersoni
|
Mammals
|
Western US
|
Europe and North America
|
[56][57]
|
Ehrlichiosis
|
Ehrlichia chaffeensis and Ehrlichia ewingi
|
Amblyomma americanum
|
Mammals
|
Canada
|
North America and Europe
|
[58][59]
|
Leptospirosis
|
Leptospira spp.
|
Environmental transmission
|
Mammals
|
Japan and Europe
|
Asia, Australia, America and South Europe
|
[60][61]
|
Lyme disease
|
Borrelia burgdorferi
|
Ixodes scapularis
|
Rodents
|
USA
|
North America and Eurasia
|
[62][63][64]
|
Powassan virus disease
|
Powassan virus
|
Several tick species
|
Mammals
|
Unknown
|
North America
|
[65]
|
Q fever
|
Coxiella burnetii
|
D. reticulatus
|
Ruminants (cattle, goat and sheep)
|
Australia
|
Europe and North America
|
[66]
|
Rocky Mountain spotted fever
|
Rickettsia rickettsii
|
D. andersoni
|
Mammals
|
South and Central America
|
North America
|
[67]
|
Rickettsia parkeri
|
A. maculatum
|
Central and North America
|
On the other hand, although vector-borne diseases are prone to be impacted by global warming, other kinds of weather events (such as abundant rains, extreme drought or changes in air currents) may play an important role in their geographical expansion. The visual impact becomes particularly evident when we examine the following NASA simulation (
https://www.youtube.com/watch?v=h1eRp0EGOmE). It demonstrates how alterations in air currents caused by extreme events such as hurricanes can influence the dispersal of particles and microorganisms to new regions of the world. These events have been extensively studied in processes such as El Niño events. After these extreme weather events, an increase in diseases, like Rift Valley fever, has been observed. This acute viral hemorrhagic fever is caused by a member of the genus
Phlebovirus. It triggers high livestock morbidity and mortality and has traditionally been identified in sub-Sahara regions. However, there have recently been outbreaks as far north as the Arabian Peninsula and Asia, with the potential to spread further into Europe in the future
[68][48][69].
The ability of microbial pathogens to mutate and adapt to environmental changes is a significant factor in understanding the potential impact of climate change on livestock. For instance, in the case of Venezuelan equine encephalitis virus (VEEV), a single mutation allowed it to adapt to a new vector after a shift in mosquito populations due to deforestation. This highlights the rapid response of viruses to changes in their vector populations
[49]. In addition, RNA viruses have no proof-reading, leading to a high evolutionary rate, mainly those with segmented genomes. While not directly affected by climate conditions, climate change could reduce the species barrier and facilitate contagion to new species through the invasion of new habitats
[49].
Finally, apiculture is a livestock sector often forgotten when it comes to climate change.
Apis mellifera is the most common species of honeybees in Europe and is the most valuable pollinator of agricultural crops worldwide
[70][71][72]. However, they suffer from a wide variety of bacterial, fungal and viral pathogens, as well as microsporidial or ectoparasitic mites. Among its most common diseases, European foulbrood (EFB), American foulbrood (AFB), chronic bee paralysis (CBP), sacral brood, calcareous brood and varroosis
[73] are worth mentioning. Models have shown a positive relationship between temperature and the incidence of some of these important diseases
[74]. Two of the most studied ones are chronic bee paralysis (caused by an unclassified bipartite RNA virus usually called chronic bee paralysis virus)
[75] and American foulbrood (caused by the Gram-positive bacteria
Paenibacillus larvae), whose expansion and intensification have already been reported despite the absence of models linking it to climate change
[73][76].
4. Fishing and Marine Microbiome
Fishing and shellfish are relevant food industrial sectors. On average, a person eats approximately 20.5 kg of fish and shellfish every year. The total seafood from 2018 landings had a farm-gate production value exceeding USD 401 billion
[1][77]. However, marine animals are mainly ectotherms, and, as a result, they are particularly sensitive to changes in water temperature, which highlights the effect of global warming on this fauna. European seas have seen a significant temperature increase, disrupting migration patterns, reproductive cycles, food availability, and species distribution
[78].
Climate change may also affect other environmental factors such as seawater acidification, hypoxia, CO
2 accumulation, salinity or sea level modifications
[79] (
Figure 2). These changes disturb marine ecosystems, including microbial biodiversity, which forms the basis of marine food webs and is responsible for important biogeochemical cycles
[80]. However, most of the ocean observation programs and studies do not target microbes
[3][81], and the few reported just point to changes in the ocean’s microbial biodiversity mainly due to the increase in water temperatures and sea acidification
[82][83]. Therefore, Wang and co-workers
[84] observed that warmer oceans will alter microbial communities, especially in thermally stable regions, and Hutchins and Fu
[85] reported that ocean warming causes losses in microbial populations, leading to the predominance of a few earlier insignificant taxa.
Figure 2. Main effects of climate change on key chemical and physical factors influencing the community composition of marine planktonic microorganisms. The current ocean (a) vs. the future one (b) after 2100. Among the changes that will have the greatest impact are (i) increases in atmospheric CO2 uptake and, therefore, water acidification, (ii) increase in temperature, (iii) shallowing of the surface mixed layer, (iv) intensified stratification, leading to lower vertical fluxes of nutrients, and (v) reduction in oxygen both at the surface and in the underlying deep ocean. All these changes lead to changes in microbial biodiversity and expansion of both pathogen geographic ranges and pathogen virulence, inducing an increase in disease outbreaks and fauna and flora mortality.
To date, 25 viruses, 33 bacteria, 23 protists and 21 metazoans have been reported as the main causes of marine diseases in plants, corals, mollusks, crustaceans, echinoderms, fishes, turtles and mammals
[79], although it is estimated that a greater part of the diversity in marine microbial biodiversity remains undiscovered, with less than 0.1% being described
[86]. Thus, some studies have illustrated how marine warming alters host–pathogen dynamics, which can be described as follows:
-
First, environmental changes like those caused by climate change may lead to stress in both fish and shellfish species, leading to lower immune responses against various pathogens and diseases
[3]. Urchins have shown a strong correlation between mass mortality events and long-term elevated temperatures
[87].
-
Second, a rise in temperatures leads to an increase in several marine pathogens’ virulence by increasing their metabolism and inducing higher rates of transmission
[87]. Such as the case of the host–pathogen interaction between
Pocillopora damicornis and
Vibrio coralliilyticus. At temperatures above 27 °C, pathogen virulence increases and cause lysis and mortality in
P. damicornis [88].
-
Third, pathogen geographical expansion comes with increasing temperatures. A shining example is
Perkinsus marinus, a protist parasite of the eastern oyster
Crassostrea virginica that has expanded its range due to increased winter water temperatures
[89][90
On the one hand, although temperature is presented as the dominant climate change effect disrupting microbial marine communities, there are physical and chemical factors that may also affect infection and disease transmission in aquatic environments. A reduction in water salinity has the potential to significantly impact the immune response of host organisms. For instance, oysters exposed to low-salinity stress exhibited increased susceptibility to infection by
Vibrio alginolyticus [92]. Similarly, ocean acidification can affect the immunological defenses of diverse organisms, such as urchins, mussels, oysters, finfishes, corals, bivalves and seagrasses. In addition, different studies have reported that
Vibrio tubiashii grows better and increases its infectivity against mussels (
Mytilus edulis) and hemocytes when exposed to low-pH conditions
[79][93]. On the other hand, elevated partial pressures of CO
2 (usually known as hypercarbia) may impact the immune response by activating the complement system, activating the inflammatory response and down-regulating IgM expression. Thus, the joint effect of hypercarbia and temperature changes can alter the first and second lines of finfish defense, affecting their susceptibility to infections and diseases
[79].