Perovskite-type oxides (ABO3) are a highly versatile class of materials. They are compositionally flexible, as their constituents can be chosen from a wide range of elements across the periodic table with a vast number of possible combinations. This flexibility enables the tuning of the materials’ properties by doping the A- and/or B-sites of the base structure, facilitating the application-oriented design of materials. The ability to undergo exsolution under reductive conditions makes perovskite-type oxides particularly well-suited for catalytic applications. Exsolution is a process during which B-site elements migrate to the surface of the material where they form anchored and finely dispersed nanoparticles that are crucially important for obtaining a good catalytic performance, while the perovskite base provides a stable support. Recently, exsolution catalysts have been investigated as possible materials for CO2 utilization reactions like reverse water–gas shift reactions or methane dry reforming.
An overwhelming majority of published studies on the topic agree that current global warming is a consequence of human-caused (anthropogenic) climate change
[1]. The level of the greenhouse gas CO
2 in the atmosphere—which is the main cause of anthropogenic climate change—rose sharply between the beginning of the Industrial Era (approximately 280 ppm) and today (more than 400 ppm), thus straining the carbon budget (i.e., the amount of carbon that can be released in the atmosphere while still limiting global warming to a given temperature difference)
[2]. Therefore, efforts are being undertaken to reduce CO
2 to achieve net zero emissions (e.g., the European Green Deal
[3] aims for climate neutrality of the European Union by 2050).
Closing the carbon cycle via CO
2 conversion is a possible way towards meeting this goal. This means no longer treating emitted CO
2 as a waste gas but as a readily available carbon source for base materials or fuels. A schematic depiction of such a closed carbon cycle in industries is shown in
Figure 1. Flue gas from industries and power generation is captured and converted. The products can be used as feedstock in the chemical industry or as fuels which can be stored for later use. CO
2 conversion can be driven in different ways. In a 2019 review, De Luna et al. discussed the question of how CO
2 electrolysis (i.e., electrochemical processes) could be a pathway to replacing the chemicals and fuels produced petrochemically
[4]. In the authors’ own work, we investigate a different approach to achieve CO
2 conversion. Instead of electrolysis, seemingly straightforward reactions like the reverse water–gas shift reaction (rWGS, Equation (1)) or the dry reforming of methane (DRM, Equation (2)) are used to directly transform CO
2 into CO or syngas (a mixture of CO and H
2), respectively.


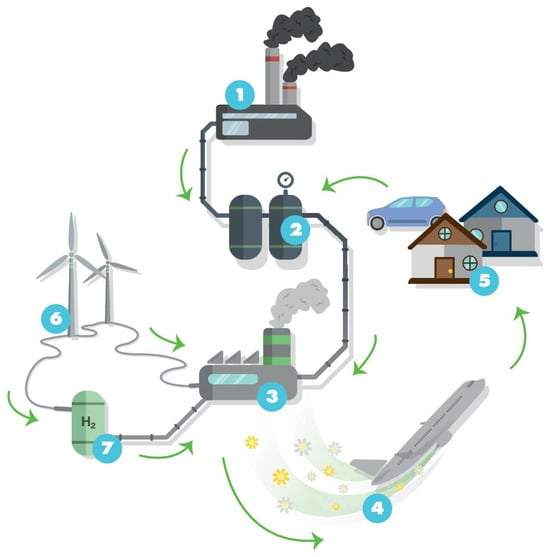
Figure 1. Closing the carbon cycle: CO2 from flue gas emitted from industries (1) is captured (2) and converted (3) into fuels (e.g., for aviation, (4)) or feedstocks for the chemical industry. Produced fuels can be stored or used (e.g., for transportation or heating, (5)) the CO2 set free here is then recycled and used for another conversion. To operate conversion sustainably, renewable energy (6) is used both directly during the conversion and indirectly during the production of green H2 (7), which is needed, for instance, in rWGS reactions.
Due to its stability, reactions using CO
2 as a raw material necessitate the use of a catalyst for activation. Furthermore, CO
2 activation processes demand large amounts of energy. This opens up energy storage as a second potential application of CO
2 conversion (apart from the reduction of emissions), as electrical energy (ideally renewably generated with solar panels, wind turbines, geothermal plants…) is converted into chemical energy of fuels that can be stored more easily.
For both rWGS and DRM, a large number of diverse catalytically active materials have been extensively researched, for instance, supported catalysts based on noble metals
[5][6] or Ni
[7]. However, there are drawbacks for these catalysts, e.g., Ni-based catalysts are vulnerable to deactivation via carbon deposition
[8] and catalysts using noble metals, while not as susceptible to coking
[9], are expensive. Additionally, high temperatures are required when performing rWGS or DRM, which can cause nanoparticles to sinter together, thus reducing the catalytically active surface. This means that suitable catalysts for large-scale applications need to be stable at high temperatures, resist coking, and contain catalytically active elements that are available at the surface (e.g., as nanoparticles, which should not be prone to sintering in order to maintain their activity). A promising class of materials for use as such CO
2 conversion catalysts are perovskite-type oxides due to their stability at high temperatures, their compositional flexibility, and their ability to undergo exsolution:
Perovskite-type oxides share the general formula ABO
3, where A and B are two cations of different sizes that are arranged as shown in
Figure 2a. The larger A-site cations make up the corners of the unit cell; the smaller B-site cations fill the center of the cell and are octahedrally coordinated by oxygen atoms. Depending on the differences in the atomic radii of the cations, cubic, orthorhombic, or rhombohedral cells can occur. A- and B-site elements can vary over a wide range of the periodic table, paving the way to a vast number of possible materials. Moreover, both sites can be doped, which not only introduces an even larger number of possible materials, but also facilitates a material design approach, as the materials’ properties can be tuned with the specific applications in mind
[10]. Aside from catalysis, applications of perovskite-type oxides include, for instance, electrodes used in electrochemistry or fuel cells
[11][12][13].
Exsolution is a striking property of perovskite-type oxides that causes their particular potential as catalysts
[14], especially with respect to the requirements of finely dispersed and sinter-resistant nanoparticles. Exsolution is an extensively studied process
[15][16][17], during which the surface of the perovskite is decorated by nanoparticles made up of B-site elements (schematically shown in
Figure 2b): Under reducing conditions, B-site cations move to the surface of the material, where they form finely dispersed and well anchored nanoparticles. The make-up (oxidic vs. metallic composition) of these nanoparticles can be tuned by the composition of the perovskite (influenced by doping)
[10] as well as the exsolution conditions
[17], thus allowing even more control of the specific catalytic properties of the material.
Figure 2. “(
a) The perovskite structure and (
b) a schematic depiction of the exsolution process. In a wet hydrogen atmosphere and under heat treatment, additional oxygen vacancies are created, and dopant atoms reduce, resulting in their migration from the bulk to the surface, where they form nanoparticles.” Reprinted from
[18] under CC-BY 4.0.
This entry aims to showcase how perovskite-type oxides as catalysts in CO2 conversion processes are investigated to assess their suitability.