1. Introduction
Iron-dependent proteins play a central role in oxygen transport, electron transport, redox reactions, and nucleotide synthesis. Iron is typically incorporated into proteins as heme, iron sulfur clusters, or directly into tertiary structure cavities
[1]. As there is a heavy demand for iron in order to maintain normal metabolic processes, iron is efficiently conserved in the mammalian body. Indeed, mammalian iron excretion is highly controlled, and the iron content of sweat and urine is low. It has been estimated that once absorbed, the average iron atom spends approximately 10 years inside a human before being excreted. Thus, iron is located in a range of intracellular pools, some relatively kinetically inert such as ferritin and others, such as the “labile iron pool” (LIP), which is labile, thereby facilitating the transmembrane movement of iron and iron donation to apoenzymes. Bioavailable, cytosolic iron exists in the iron(II) (ferrous) state and loosely interacts with a range of counter ions
[2]. Williams argued that the electrode potential of the cytosol favours iron(II) over iron(III), and because the iron(II)-binding constants for many cytosolic enzymes fall in the range 10
−8–10
−7 M
[3], it requires a similar standing concentration of iron(II) in order to prevent dissociation from the enzyme. Fluorescent probe studies, using calcein
[4] and Phen Green SK
[5], have demonstrated that the majority of the cytosolic LIP is iron(II), which, in view of the 100-fold higher kinetic lability of iron(II) when compared with iron(III)
[6], is a logical strategy. Iron(II) is generally the form involved in intracellular translocation, for instance, incorporation into iron-requiring enzymes, incorporation into ferritin, and transport across membranes by the divalent metal transporter 1 (DMT1) and ferroportin. This kinetically labile group of iron species, LIP, is estimated to exist in concentrations of the order of 1 µM
[2].
Iron(II) is capable of forming a range of oxygen radicals under aerobic conditions, via Fenton chemistry
[7,8][7][8] and so the level of LIP needs to be carefully controlled, thereby limiting the potential toxicity of this critically important iron pool. In mammals, cellular LIP homeostasis is achieved by two “Iron Regulatory Proteins” (IRP 1 and IRP 2), which control the iron uptake into the cell via transferrin receptor 1, as well as iron export via ferroportin and iron storage in ferritin. Both IRP 1 and IRP 2 are RNA-binding proteins, capable of binding to ‘iron response elements’ (IREs) on mRNA
[9]. By binding to IREs they inhibit the translation or degradation of mRNAs, which encode proteins involved in iron storage and iron import, thereby influencing LIP levels
[10]. With IRP 1, IREs compete for binding to the protein with the coenzyme 4Fe-4S cluster of aconitase (ACO 1). With IRP 2, the insertion of a di-iron centre into the F-box and leucine-rich repeat protein 5 (FBXL5) permits the formation of a ubiquitin ligase complex, which subsequently binds to IRP 2, inducing its breakdown by the proteasomal pathway. Thus, the assembly of these two iron centres, namely 4Fe-4S and Fe-O-Fe, creates an integrated iron sensing mechanism for LIP, which in turn is linked to many feedback loops.
2. Chemical Components of LIP
Many ligands previously associated with the cytosolic LIP chelate iron(III) but not iron(II) at pH 7.0; namely, amino acids
[12][11], ATP/AMP
[13][12], and inositol phosphates
[14][13]. In contrast, citrate does bind iron(II) under cytoplasmic conditions and has been suggested to be the major component of LIP
[15][14]. Using the logK
1 value for iron(II) of 5.12
[17][15], GSH is predicted to bind iron(II) to a considerable extent at pH 7.0. GSH also rapidly reacts with
hexaaquo·iron(III) converting it to
hexaaquo·iron(II) (
1) (
Scheme 1)
[20][16].
Scheme 1. Structures of the aqueous, glutathione, and glutathione/carnosine complexes with iron(II). (1) hexaaquo·iron(II); (2) iron(II)glutathione; (3) iron(II)glutathione·carnosine; (4) carnosine.
GSH does not chelate iron(II) as, unlike cysteine, the bidentate coordination would require the formation of chelate rings containing either 9, 10, or 11 atoms. Such ring sizes are associated with unfavourable entropy differences. The non-chelating structure (
2) (
Scheme 1) agrees with that previously proposed for glutathione coordination of metal ions
[21][17].
Over the concentration ranges of GSH (2–10 mM) and iron(II) (0.5–5 µM), the two major cytosolic iron(II) species are reliably predicted to be Fe
2+·GS (
2) and
hexaaquo·Fe
2+ (
1), with the glutathione complex dominating. The concentration of [Fe(H
2O)
6]
2+ (
1) is linearly related to the total concentration of cytosolic iron(II) and is predicted to vary between 10
−8 M and 5 × 10
−7 M for a range of total iron(II) concentration between 1 µM and 10 µM.
As iron(II)glutathione, under physiological conditions, exists as a hydrated iron(II) cation with a single coordination site being occupied by monodentate glutathione (
2), other low-molecular-weight ligands present in the cytosol can simultaneously coordinate to this ion, forming a ternary complex. The additional bidentate or tridentate ligand would need to possess a similar affinity for iron(II) as that of GSH in order to form an appreciable concentration of the ternary complex; the ligand would also need to be present at a relatively high concentration (1–10 mM). These requirements severely limit the number of such possibilities. Significantly, a group of widely distributed histidine-containing dipeptides, including carnosine (
4) (
Scheme 1), are capable of binding transition metals
[24][18].
Thus, LIP is likely to consist of several iron(II) complexes in equilibrium with each other (
Scheme 2), with glutathione acting as a redox buffer locking iron into the iron(II) state. The concentrations of iron(II), glutathione, and carnosine will influence the relative concentrations of the LIP components (
1), (
2), and (
3).
Scheme 2.
Equilibria between iron(II) (
1
), glutathione (GSH), and carnosine (
4
).
3. The Nature of the Labile Iron Pool in Different Intracellular Organelles
Mitochondrial Labile Iron Pool. Mitochondria are major sites for heme and iron-sulfur cluster synthesis in both plants and animals and, consequently, there is a constant iron influx into mitochondria as they export both heme and iron-sulfur clusters for use elsewhere in the cell. There are almost certainly multiple mechanisms for mitochondrial iron uptake.
Lysosomal Labile Iron Pool. The lysosome is involved in the breakdown of many iron-containing proteins, in particular ferritin. The digestion of ferritin generates a pool of ferric iron, which, when exposed to the acid pH range of most lysosomes (pH 4.5–5.5), will be complexed by citrate. Citrate levels in the lysosome typically fall in the range of 10–20 mM. Glutathione has a relatively low affinity for iron at pH 5.0 and so the concentration of iron(II)glutathione is predicted to be much lower than that found in the cytosol.
Nuclear Labile Iron Pool. The sequestering of genetic material within the nucleus of the eukaryotic cell provides the nucleus with the ability to regulate gene expression. Iron is an essential component for the expression of many genes
[47,48][19][20] and of multiple enzymes involved in DNA metabolism
[49][21], including both DNA synthesis and repair. Iron-dependent enzymes include DNA polymerase and primase, DNA-helicases, nucleases, glycosylases, and demethylases together with ribonucleotide reductases, many of which contain iron sulfur clusters
[49][21].
4. Isolation and Characterization of Labile Iron Species
It should be clear from the previous discussion in this overview that in all probability, the LIP consists of a number of components and that these may differ between intracellular compartments by virtue of different pH values and concentrations of various iron-binding compounds in these various compartments. In the majority of compartments, namely cytosol, the mitochondrion, and the nucleus, the dominant redox state is iron(II), which is kinetically labile. This property renders it extremely difficult to isolate and purify such species from any biological matrix. Chromatography will not be possible due to the lability of iron(II)glutathione; iron dissociates from the complex and binds to other competing ligands including the stationary phase of the column. Mass spectrometry would appear to be the most promising method for the identification of labile iron species in biological matrices
[52][22].
5. Iron-Sensitive Fluorescent Probes
The design of iron probes is typically based on bifunctional structures containing both a chelating agent and a fluorophore. A third function can be incorporated into the probe in order to influence subcellular localization
[53,54][23][24]. For iron, the chelating agent can be selective for either iron(II) or iron(III). Probes to detect iron may contain either “turn-on” fluorophores, in which the binding of iron increases fluorescence, or “turn-off” fluorophores, in which the binding of iron quenches the fluorescence
[55][25].
With ‘turn off’ probes, the concentration of LIP is determined by the decrease in fluorescence after correcting for photobleaching and leakage of the probe using either an
in situ or an
ex-situ calibration method
[5,57][5][26]. Based on Equation (1), the equilibrium constant (K
probe) can be used to quantify LIP, where LIP = [Fe
2+] + [Fe
2+·probe]. In principle, K
probe, [Fe
2+·probe], and [probe] can be determined. In order to minimise the efflux from the cell, the acetoxy methoxy group (AM) has been successfully introduced. AM enhances the membrane permeability of the probe, but cytosolic esterases rapidly cleave the AM esters to the more hydrophilic carboxylate function (Equation (2)).
Rho Nox-1, an interesting iron(II) probe, has been investigated for the fluorescent-based detection of LIP
[59,60][27][28]. The Fe
2+-mediated deoxygenation of the N-oxide group on the fluorophore is reported to lead to an enhanced fluorescence, and as such, it is a “turn-on” probe, Equation (3). The mechanism of this deoxygenation reaction is not clear at the present time, and the reduction in the N-oxide may also be facilitated by thiols, including glutathione, which is present in the cytosol at high concentrations. More control studies are required before this class of probes can be reliably used to report LIP concentrations.
Cytosolic Probes. There are many fluorescent probes, selective for either iron(II) or iron(III), that have been previously described
[61,62][29][30]. However, the properties of many of these probes have not been carefully investigated in cell culture systems. Probes that have been investigated in some detail are disscussed, namely calcein-AM (
6), Phengreen SK diacetate (
7), Fura-2AM (
8), Rho Nox-1 (
5), and Cytosense LI
TM.
Calcein-AM (
6) is currently the most widely adopted fluorescent iron probe, having been studied in a wide range of cells
[63,64][31][32]. Typical behaviour is presented in
Figure 41 where a single HepG2 cell is monitored
[65][33]. As iron(II), presented as iron(II) ammonium sulfate, enters the cytosol it quenches the calcein fluorescence. Upon the addition of Salicylaldehyde isonicotinoyl hydrazone (SIH,
9) (40–100 µM), the chelator gains rapid access to the cytosol where it out-competes calcein for iron(II) and consequently the fluorescence rapidly increases. When the behaviour of SIH (
9) and deferoxamine (DFO) are compared in the presence of another cell line, it is clear that DFO fails to gain entry to the cytosol during the time scale of the study
Figure 52 [63][31].
Figure 41. LIP levels in a single human hepatoma HepG2 cell. Iron(II) ammonium sulfate (FAS, 20 µM) was added to half of the cells. SIH (100 µM) was added after the indicated time interval
[65][33].
Figure 52. Dequenching of calcein–iron complexes in K
562 cells by extracellularly added chelators (100 μM) at zero time. Arrow indicates the addition of SIH (100 μM)
[63][31].
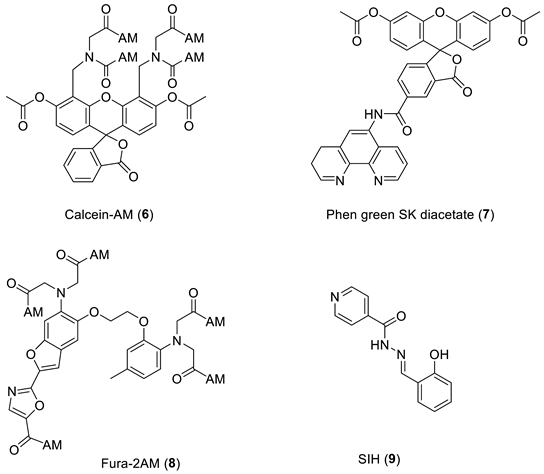
As indicated by the discussion centred on Equation (1) using such probes, it is, in principle, possible to determine the concentration of LIP. The concentration of
6 can be determined from the fluorescence of a single cell or a suspension of cells. [Fe
2+·
6] is measured from the change in fluorescence upon the addition of SIH and the binding constant of iron(II) to the probe.
Despite this limitation, calcein has been utilized extremely effectively to provide information on changes in LIP levels in a range of cell types. Thus, the rate of influx of iron chelators into cells can be directly compared
[67][34] (
Figure 63), the UVA-induced production of LIP can be monitored
[68][35] (
Figure 74), and the influence of LIP on the erythrocyte stage of
Plasmodium falciparum can be monitored during cell culture (
Figure 85)
[69][36].
Figure 63. Dequenching of calcein–iron complexes in K562 cells. Various chelators (100 μM) added at different time points, SIH (100 μM) marked by *. The chelators 102, 41, 165, and 117 are analogues of Deferiprone (DFP)
[67][34].
Figure 74. UVA-induced production of LIP in skin fibroblasts. EC—epicatechin (30 μM) [68]. * = significant difference. UVA-induced production of LIP in skin fibroblasts. EC—epicatechin (30 μM) [35]. * = significant difference.
Figure 85. LIP levels in various malaria parasite stages. The LIP increases as the parasite matures within the host red blood cell. The LIP of uninfected and different-stage parasitized RBCs was determined by assessing the fluorescence of calcein-loaded cells with the addition of the iron chelator, deferiprone (DFP)
[69][36]. * = significant difference.
Phen green SK diacetate (
7) is metabolized by cytosol esterases to Phen green SK (
10), as shown in Equation (4), and hence is largely trapped in the cytosol. However, a small proportion of
10 gains access to the mitochondria and lysosomes
[70][37]. Phen green SK diacetate (
7) has been utilized to measure cytosolic LIP by using an
ex-situ calibration method
[70,71][37][38]. When this method was applied to isolated rat hepatocytes, a range of LIP values was obtained (
Figure 96), with a large proportion falling in the range of 0.8–2.0 µM
[71][38].
Figure 96. Frequency distribution of the hepatocellular concentration of LIP. Cells were incubated with PG SK diacetate (20 μM) for 10 min. Bipyridyl (5 mM) was added and the resulting fluorescence was noted
[71][38].
Rho Nox-1 (
5) is reported to be selectively sensitive to the presence of iron(II)
[59][27], and confocal fluorescence microscopy images of HepG2 cells incubated with (NH
4)
2Fe(SO
4)
2 register an increase in fluorescence intensity (
Figure 107). As indicated in the earlier section on “Iron-sensitive fluorescent probes”, more control studies on this group of probes are urgently required. Stability studies are reported for coincubation with GSH, but only at 1 mM, although cytosolic GSH is at least 8 mM. It has not been established whether Rho Nox-1 (
5) can detect iron(II) in the presence of physiological levels of GSH.
Figure 107. Quantification of LIP in HepG2 cells supplemented with (NH
4)
2Fe(SO
4)
2 (100 µM) for 30 min at 37 °C. 2,2′ Bipyridyl (Bpy, 1 mM) was used to reduce the availability of iron(II)
[59][27].
Hydroxypyridin-4-one (HOPO)-containing probes have been investigated for the quantification of LIP
[54][24]. Under biological conditions, they are selective for iron, binding iron(II), and rapidly autoxidizing the metal to iron(III) (Equation (5)) in a similar fashion to that of DFO. CP655 (
11) achieves rapid entry into cells without the necessity of using AM or acetyl esters.
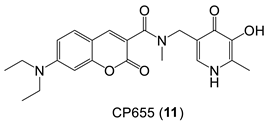
Mitochondrial Probes. As mitochondria are the main utilizers of iron in the cell, as well as being a major source of the superoxide anion, O
2−•, iron homeostasis is critically important. An increase in the mitochondrial iron burden is likely to be linked to associated pathology, for instance with Friedreich’s ataxia where there is inefficient iron sulfur cluster synthesis
[76][39].
By taking advantage of the substantial negative electrochemical potential maintained across the inner mitochondrion membrane (typically −200 mV), delocalized cations are able to cross the membrane and hence be accumulated with a distribution driven by the Nernst potential, typically in excess of 1000-fold. Triphenyl phosphonium salts (
12) are such examples where the positive charge formally associated with the central phosphorus atom is delocalized over the three phenyl rings, resulting in a low electron density. This, together with the overall lipophilic character of the molecule, enables rapid penetration of membranes. This useful property of lipophilic cations has been utilized to target fluorescent iron sensors in the mitochondrion. Both Petrat’s
[77][40] and Cabantchik’s groups
[78,79][41][42] have utilized a derivative of rhodamine (RPA,
13) to monitor mitochondrial iron levels.
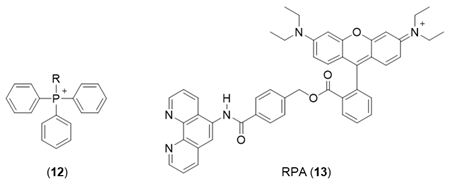
By incorporating an iron-chelating moiety into the molecule (
14), it becomes possible to monitor the mitochondrial LIP. BP19 (
14) has been utilized to monitor the mitochondrial LIP of cultured fibroblasts obtained from Friedreich’s ataxia patients
[81][43]. The mean levels of mitochondrial LIP in Friedreich’s ataxia cells were found to be 1.11 ± 0.37 µM, whereas those from healthy donors were 0.17 ± 0.12 µM. This finding supports the observation of Rouault et al.
[82][44] that there is an accumulation of redox-active labile iron in the mitochondria of Friedreich’s ataxia patients.
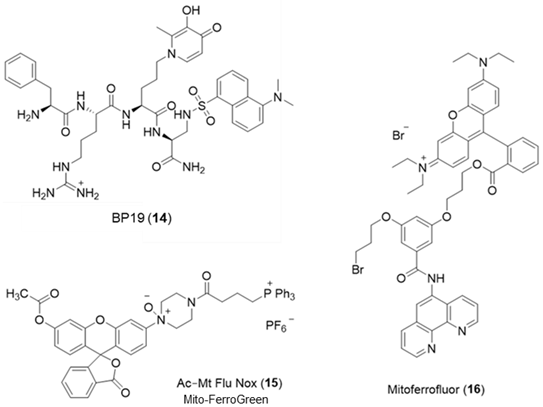
Lysosomal targeting Probes. The endosomal/lysosomal compartment of cells contains a relatively high level of labile iron, largely resulting from the breakdown products of both mitochondria
[85][45] and ferritin
[86][46]. Chelation of this iron pool protects against oxidative stress-induced cellular damage
[46,87][47][48].
The hydroxypyridinone SF34 (
17) has been demonstrated to only be located in endosomal/lysosomal compartments in macrophages
[89][49]. SF34 does not permeate membranes rapidly and so can be trapped within the endosomal/lysosomal compartment, which it enters by endocytosis.
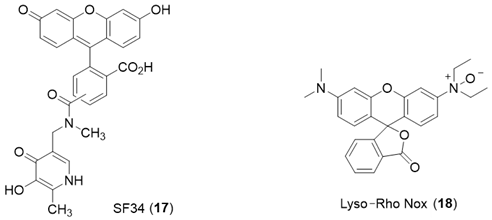
The
Rho Nox-1 analogue,
Lyso-Rho Nox (
18), has been reported to be localized in the lysosomal compartment of human adenocarcinoma (MCF-7) cells, and this property has been utilized to report differences in lysosomal LIP levels
[91][50].
6. NIR Probes
The development and application of near-infrared (NIR) technologies is also under investigation, in order to facilitate our understanding of the nature of cytosolic iron pools
in vivo. This is primarily due to the unique properties of NIR light and its interaction with biological tissues. Namely, by exploiting a region of the electromagnetic spectrum, which is less absorbed and scattered (i.e., above 650 nm), NIR light is known to offer several advantages, such as increased tissue penetration, limited autofluorescence by endogenous molecules, and, thus, minimized background interference, along with reduced photobleaching of the fluorophores and photodamage to the tissues.
In the context of iron(II)-targeted imaging, Zheng and collaborators developed the fluorescent probe DCI-Fe(II) (
19), which can sense the metal on the basis of
N-oxide chemistry
[107][51], being reported to be highly effective in the detection and imaging of iron(II) ions in real-time, both in cells and
in vivo. In particular, this agent demonstrated a metal concentration-dependent NIR signal turn-on response (at 700 nm) coupled to a large Stokes shift (195 nm), along with a detection limit of 51 nM for iron(II). Additionally, a far-red probe (i.e., 620 nm emission) was reported as an effective imaging probe for the detection of Fe(II) levels in mouse models of inflammation
[101][52].
7. Conclusions
The potential of using iron-sensitive probes for the quantification of LIP is considered, and a range of applications of such probes is described. The quantification of LIP by the use of iron-specific fluorescent probes is currently under development, but it is now possible to monitor LIP levels in cytosolic, mitochondrial, and lysosomal pools of cells in suspension. Such measurements made under in vivo conditions will be more difficult, but NIR probes are under development for this purpose. Undoubtedly, the ability to visualize and quantify iron dynamics in living organisms is expected to have a significant impact on the development of targeted therapeutic interventions for iron-related pathological conditions.