Bisindolylmaleimides (BIMs) are widely recognised for their activity against protein kinases and from a synthetic perspective can be highly functionalised or chemically manipulated. This provides the opportunity to generate novel analogues and derivatives with unique biological profiles. Although BIMs show significant activity themselves and serve as targets in their own right, they are also important precursors in the synthesis of the indolocarbazole compound class.
2. Bisindolylmaleimides (BIMs) and BIM-Type Inhibitors
Arcyriarubin A (
BIM-IV) is the simplest bisindolylmaleimide that belongs to the family of pigments, arcyriarubin A-C (
Figure 2). It was isolated from the
Myxomycetes slime moulds by Steglich et al. in 1980
[9][2].
BIM-IV was a potent sub-micromolar inhibitor of protein kinase C and exhibited micromolar inhibition against seven of the other PKC isoenzymes. Fabre et al. investigated the influence of the maleimide headgroup on
BIM-IV against PKC and PKA by comparison with the succinimide
79 and the lactam derivative
80 [38][3]. Both
79 and
80 were determined to have low inhibitory activity compared to
BIM-IV against both PKC and PKA (
Figure 2).
Figure 2.
Kinase inhibition of Arcyriarubin A (
BIM-IV
) and derivatives.
In subsequent studies, lactam
81 exhibited more potent activity against PKC than against PKA in micromolar concentrations
[19,39][4][5]. These studies reveal the critical nature of the maleimide ring to the kinase inhibition of arcyriarubin A (
BIM-IV). Additional antimicrobial screening of
BIM-IV revealed that unlike the indolocarbazoles staurosporine and K-252a, it inhibited sporulation and inhibited the growth of
Streptomyces chartreusis and
Streptomyces griseus [40][6].
Interest subsequently shifted towards
N-alkylated indole subunits to further probe kinase inhibition. In 1990, Toullec et al. investigated the activity of the bisindolylmaleimide GF109203X (
BIM-I) against PKC and five other protein kinases (
Figure 3)
[41][7].
Figure 3.
Selective PKC inhibitor GF109203X (
BIM-I
).
GF109204X (
BIM-I) was quickly recognized as an inhibitor of specific PKC isoforms at nanomolar concentrations (
Figure 3). It was also discovered as a competitive inhibitor of ATP (
Ki = 14 nM) and it efficiently halted PKC-mediated phosphorylation and successfully inhibited collagen-triggered ATP secretion and collagen- and thrombin-induced platelet aggregation. As well as being used as a standard molecular tool to explore the role of PKC in disease, more recently GF109203X has been identified as a potent agonist of β-catenin accumulation in preosteoblast cells, promoting osteoblast differentiation and bone formation (through suppression of GSK-3β kinase)
[42,43][8][9]. Another new application was identified in 2017 in the inhibition of exosome and microvesicle release to improve the efficiency of cancer treatment
[44][10].
In 2005, Graff et al. reported the dialkylated bisindolylmaleimide enzastaurin (LY317615.HCl) (
57) as an ATP-competitive inhibitor of PKC (
Figure 4). It was found to exhibit potent activity against the PKC isoforms α, β, γ and ε, with some selectivity reported for PKC-β
[45][11]. The interaction between PKC and the phosphatidylinositol 3-kinase (PI3K)/AKT pathway is consistent with the fact that
57 interferes with AKT pathway signalling and acts through mechanisms with both direct and indirect antitumour effects, such as the direct induction of apoptosis and suppression of tumour cell proliferation, or by indirectly halting tumour-induced angiogenesis
[46][12]. In early clinical trials, enzastaurin (
57) was reported as an oral serine/threonine kinase inhibitor which selectively targets PKC-β and the PI3/AKT signalling pathways. Enzastaurin suppressed angiogenesis with a reduction in the growth of human glioblastoma and colon carcinoma xenografts
[45][11]. The drug was granted orphan drug status for the treatment of diffuse large B-cell lymphoma (DLBCL) in 2007; clinical trials were halted after limited efficacy as a monotherapy for cancer was reported
[45,47][11][13].
Figure 4.
Enazastaurin (
57
) and its inhibitory profile against PKC.
Having completed phase III, no toxicity was reported when used for the treatment of solid tumours and hematologic malignancies involving >3000 patients, and thus enzastaurin has a well-mapped safety profile. This has led to a focus on drug repurposing and licensing for specific uses under various names (ENZA, DB102, AR101 and Kinenza). In 2020, enzastaurin was granted Fast Track designation by the FDA for the treatment of newly diagnosed glioblastoma multiforme with biomarker DGM1 (ENGINE clinical trial in combination with R-CHOP and the ENGAGE clinical trial with Temozolomide), and in 2022 it was granted orphan drug status in the US and safe-to-proceed through the PREVEnt clinical trial for patients with COL3A1 mutation with vascular Ehlers–Danlos Syndrome
[48,49,50,51][14][15][16][17]. It is clear that Enzastaurin has a clinical role to play in future.
Expanding on the theme of
N-alkylation, macrocyclic bisindolylmaleimides were investigated (
Figure 5). Initially, the
N–N′ bridged alcohol
60 (LY326449) was prepared
[32][18]. Although there was a noticeable improvement in activity against PKC-α and PKC-β
2 and it showed >10,000-fold selectivity for PKC over PKA, it did not progress to the clinic
[52][19]. Subsequent incorporation of dimethylamine led to the important discovery of ruboxistaurin (LY333531) (
61). This compound was found to be a potent and selective nanomolar inhibitor of PKC-β
2. Given these significant results, the activity of
61 was evaluated against other PKC isoforms, but potent inhibition was only reported for PKC-β
1 (IC
50 = 0.0047 μM)
[53][20]. It was found to interact at the ATP binding site to disrupt the phosphotransferase activity of novel and conventional PKC isoforms
[54][21].
Figure 5.
Ruboxistaurin (
61
) and its derivative
60
.
Further studies with
61 revealed that PKC activation of the β isoenzyme, in particular, contributes to vascular complications, including hemodynamic changes associated with diabetes, and thus PKC-β inhibition may be a promising approach. The efficacy of ruboxistaurin was evaluated for an anticancer effect as a combination therapy, which led to the enhanced activity of paclitaxel but also carmustine and when co-administered with
61 in separate assays. It progressed to phase 1 oncology trials and phase 2/3 for diabetic neuropathy, with no further progress reported after 2006, and a withdrawal report was issued in 2007
[55,56,57][22][23][24]. However, as with enzastaurin, artificial intelligence has identified new applications and ruboxistaurin is currently under evaluation by Dermbiont (as DBI-102, a topical application for hyperpigmentation) and Recursion (as REC-3599 for the treatment of Tay–Sachs disease or GM2 gangliosidosis)
[58][25].
Kuo et al. investigated the inhibition of PKC-γ in order to access new therapies for chronic pain
[33][26] and macrocyclic bisindolylmaleimides that mimic ruboxistaurin (
61) with the incorporation of the 7-azaindole moiety were reported (
Figure 6). Initially, the bisindole system and mono-7-azaindole derivatives were prepared and evaluated. Following this, bis-7-azaindolylmaleimide was generated with ether chains of varying lengths (n).
Figure 6.
Macrocyclic mono- and bis-azaindolylmaleimides and kinase inhibition.
Upon evaluation of their activity,
61 was still more potent for PKC-γ (IC
50 = 0.3 μM) than any of the macrocyclic candidates in this synthetic library (
Figure 6). The most interesting finding was the potency against GSK-3β at sub-micromolar concentrations. By introducing the bis-7-azaindole core (
86 and
66), the activity shifted towards exclusive GSK-3β inhibition and lead compound
66 showed nanomolar inhibition of GSK-3β (IC
50 = 0.034 μM). Although a slight extension of the macrocyclic chain retained activity, longer chains, e.g.,
88 (where n = 4), significantly diminished activity against GSK-3β.
Following further evaluation of
66, no competing activity for other protein kinases was identified, with the PKC-β
2 isoenzyme as the only exception (
Figure 7). Lead compounds
66 and
87 were further screened in a broad 51-protein kinase assay to assess their degree of selectivity. They both exhibited minimal or no inhibition of other kinases in the screen, effectively inhibiting GSK-3β activity by 100% at 10 μM and identified as potential specific GSK-3β inhibitors. A glycogen synthase (GS) assay was also conducted to compare the activity of known GSK-3β inhibitor LiCl with the two lead candidates. Both aza-compounds demonstrated greater potency than LiCl (EC
50 > 3000 μM), where values of 0.06 μM and 0.39 μM were measured for
66 and
87, respectively. The selectivity and potency demonstrated by these two aza-BIMs has led to a better understanding of GSK-3β in signalling pathways associated with GSK-3β-induced disorders and to the further development of BIM-like molecules.
Figure 7.
Kinase inhibitory profile of the macrocyclic bis-azaindolylmaleimide
66
.
3. Selected Modified Indolylmaleimides: Benzofuranylindolylmaleimides (BfIMs)
The initial incorporation of other aryl units, such as 7-azaindole, into the BIM frame proved effective in enhancing inhibitory selectivity against kinases. Benzofuran was also an attractive heterocyclic component, which was initially employed by Davis et al. to prepare the maleimide
89 [59][27]. Similar to the BIMs discussed earlier in the chapter, capping the indole nitrogen with an
N-methyl group increased potency for this novel class of benzofuranylindolylmaleimides (BfIMs). Significant PKC inhibition (IC
50 = 200 nM) was reported for this
N-methyl compound (
Figure 8).
Figure 8.
First reported benzofuranylindolylmaleimide
89
and derivatives
69
.
Over ten years later, Kozikowski et al. investigated a panel of 21 BfIM compounds and assessed their ability to inhibit GSK-3β
[34,35][28][29]. From a structural perspective, 5-, 6- and 7-substitution (R
1) on the indole unit ranged from halogens to bulky groups in order to gauge tolerance, the majority of candidates contained an
N-methyl group on the indole nitrogen (R
2) and substituents were also installed on the benzofuran (R
3) (
Figure 8). Overall, there is broad acceptance of substituents, aside from large steric bulk, and low nanomolar inhibition is seen with small halogen and H-bonding groups on the indole. On the benzofuran unit, a 6-hydoxymethyl group elicited inhibition at sub-nanomolar concentrations. Two compounds in particular were explored further, methylenedioxy
70 (710 nM) and di-halogenated indole BfIM
71, which demonstrated potent nanomolar inhibition of GSK-3β (3.5 nM). In order to visualize binding in the active site,
71 was co-crystallized with the GSK-3β kinase, as shown in
Figure 9. The conformation of compound
71 in the active site is not planar, but rather, two heteroaromatic units that are orientated parallel to one another. The maleimide headgroup is confirmed to H-bond to the protein backbone of Asp133 and Val135 and given the orientation of the aromatic rings, there appears to be sufficient space for diverse substituents, as seen in the GSK-3β kinase inhibition measurements.
Figure 9.
Crystal structure of GSK-3β inhibitor
71
in the active site (PDB: 3SD0).
Further analysis of compound
71, as well as the methylenedioxy compound
70, identified the relationship between GSK-3β kinase inhibition and pancreatic cancer cell lines.
Although the methylenedioxy compound
70 was not the most potent compound, it was chosen as the clinical candidate (9-ING-41) for its broad spectrum, pre-clinical antitumour activity
[60][30]. Orphan drug status was granted for the BfIM
70 by the FDA for the treatment of neuroblastoma, as it is a potent growth suppressor of neuroblastoma cells through GSK-3β inhibition. In 2018, the FDA approved this ATP-competitive inhibitor for phase I/II clinical trials for patients with advanced cancer (clinical trial no. NCT03678883)
[61][31]. This therapeutic candidate exhibited significant activity and low toxicity in both phase I and II, which have been successfully completed.
Following this, Jeffers et al. investigated
70 as a potential treatment for bleomycin-mediated pulmonary fibrosis (PF) as myofibroblast differentiation and pulmonary fibrosis are induced by the GSK-3β signalling pathway, ex vivo and in vivo, respectively
[62][32]. It was discovered that the GSK-3β inhibitor significantly improved lung function in mice treated with TGF-β adenovirus and also bleomycin-induced PF mice models. In 2020, Anraku et al. reported a broader antiproliferative scope of 9-ING-41 (
70) against renal cancer cell lines
[63][33]. It effectively induced cell cycle arrest and apoptosis as a single agent, but also proved effective in combination with standard therapies to improve antitumour effects.
4. Selected Modified Indolylmaleimides: Napthylindolylmaleimides (NIMs)
As seen earlier, the derivatisation of the BIM frame dates back to 1992 when Davis et al. replaced one of the indole units with aryl components
[59][27]. A wide panel of compounds were generated with aryl systems, including substituted phenyls, and thienyl and pyrrolyl groups. In 2006, Peifer et al. investigated arylindolyl-2,3-maleimides and evaluated their antiangiogenic activity in an in vivo assay with chick embryos. As part of this synthetic panel, naphthyl-containing maleimides were included to assess their inhibitory potential against protein kinases (
Figure 10)
[64][34]. All compounds were screened against twelve kinases with close attention to CDKs and PKC isoenzymes. Naphthylindolylmaleimide
73 was identified as a potent inhibitor of PKC-β
1 (IC
50 = 2 nM).
Figure 10.
Naphthylindolylmaleimides
73
and
78
.
The NIMs were developed further in 2017 by van Eis et al. by modifying the naphthalene component at the 2-, 6- and 7-positions to evaluate biological activity in T and B cell proliferation assays
[37][35]. These were tested against the conventional and novel PKC isotypes, and initial assessment of the 2,6-substituted naphthalenes yielded potent PKC inhibition but little isoform selectivity. Movement of the dimethylamino methylene chain to the C7 position of the naphthalene ring (
78) yielded remarkable PKC-α (0.5 nM) and PKC-β (0.7 nM) selectivity over PKC-δ, ε, η and θ (>182 nM). Modification of the amine was detrimental to the activity and it was found that compound
78 provided an optimal balance between potency and selectivity when screened against a broad panel of 136 kinases.
5. Recent Applications of Bisindolylmaleimides and Derivatives
In recent years, non-PKC targeted effects for BIMs have come to the fore with a number of examples of repurposing existing BIMs and the development of new BIMs to align with new targets.
BIM-IX was identified by Zhang et al. to affect drug-resistant chronic myeloid leukaemia (CML) by inhibiting DNA topoisomerase and inducing cell cycle arrest and cell death
[65][36]. It appears that
BIM-IX is more effective than enzastaurin and other BIMs against BCR-ABL positive and T315I mutated cells and maintains its effect on in vivo cancer models through inhibition of topo IIa and B-Raf.
On a similar note, both Li and Winfield et al. identified new BIM compounds with diverging activity. Li identified that the active BIM
90 bound to the SH2 domain of STAT3, and that substitution of the maleimide NH with hydroxymethyl eliminated this interaction, whereas Winfield identified that kinase inhibition could be modified by its substitution to N-OH
91. In both papers, novel
N-alkylated BIMs were identified to interact with STAT3 and kinases through screening, and it is remarkable that the most active compounds contain alkyl nitrile substituents on the indole nitrogens (
90 and
91,
Figure 11)
[28,29][37][38]. Again, moving away from PKC, Mayati et al. identified inhibition of the organic cation transporter 1 (OCT1) by
BIM-IX (Ro 31-8220). This has important considerations for the activity of BIMs in drug-resistant cells and should especially be considered for other BIMs in relation to the likelihood of cellular off-target effects
[66][39].
Figure 11.
BIMs modified at the indole and maleimide nitrogens
90
and
91
.
Another example of new targets is the identification of BIMs as inhibitors of calmodulin protein
[67][40]. In this study, standard BIM compound targets are summarised as in
Table 1, and it is noted that most bioassays are conducted where the reported effects could arise from interaction with more than one molecular target. In testing a series of BIMs binding to calmodulin,
BIM II,
IV,
VII,
X and
XI were identified with nanomolar affinity and have the potential as the starting point of new calmodulin inhibitors.
Table 1. Summary of proposed targets of BIM compounds used as standards [41,59,65,68,69,70,71,72,73,74,75]. Summary of proposed targets of BIM compounds used as standards [7][27][36][41][42][43][44][45][46][47][48].
BIM-Type Ligand |
Kinase |
Family |
PDB |
Reference |
BIM-I |
PKC, GSK-3β, 5-HT3, ABCG2, OCT-1, PDK1, MSK1, MAPKAP-K1, S6K1, Chk1, PKA, DYRK1 |
BIM-II |
PKC, ABCG2, OCT-1, PDK1, MSK1, MAPKAP-K1, PKA, DYRK1 |
BIM-III |
PKC, ABCG2, OCT-1, S6K1, MAPKAPK1, RSK2, MSK1, PDK1 |
BIM-IV |
PKC, PKA, MAPKAPK1, MSK1, ABCG2 |
BIM-V |
ABCG2, SK6 |
BIM-VI |
OCT-1 |
BIM-VII |
BIM-I |
PDK1 |
AGC |
1UU8 |
[74][47] |
OCT-1 |
PIM1 |
CAMK |
1XWS |
[81][54] |
PIM1 |
CAMK |
2BIK |
[82][55] |
PIM1 |
CAMK |
2BIL |
[83][56 |
BIM-VIII |
PKC, GSK-3β, carbachol-evoked noradrenaline release, OCT-1, PDK1, MSK1, S6K1, PKA, DYRK1, MAPKAPK1 |
] |
PKC-1 |
AGC |
1ZRZ |
[84][57] |
BIM-II |
PDK1 |
AGC |
1UU7 |
[74][47] |
PDK1 |
AGC |
3ORZ |
[85][58] |
PDK1 |
AGC |
3OTU |
[85][58] |
BIM-IX |
PKC, GSK-3β, MAPKAPK1, MSK1, OCT-1, S6K1 |
BIM-III |
PDK1 |
AGC |
1UU9 |
[74][47] |
BIM-X |
Multiple Protein Kinases |
BIM-XI |
PKC, T-cell activation |
As a part of the enormous research effort to combat the COVID-19 epidemic, BIMs have also been identified to possess protective activity against SARS-CoV-2 infection. Gupta et al. identified 290 potential inhibitors from a high throughput virtual screen of 5903 molecules against seven essential coronavirus enzymes
[76][49].
BIM IX proved the most successful and the target enzyme responsible for this effect was confirmed as the protease 3CL
pro. While the compound was not as effective as ivermectin, this could prove an exciting lead compound for future studies. Following this, Huang et al. screened four PKC inhibitors:
BIM I, a derivative of BIM I (Go 6983,
Figure 12), enzastaurin and sotrastaurin for antiviral activity in a SARS-CoV-2 replicon system, and identified that all compounds reduced viral replication and all but sotrastaurin had antiviral activity against wild-type SARS-CoV-2
[77][50].
Figure 12.
Modified BIMs Go 6983 and BMA 155.
Finally, although the majority of BIM compounds maintain the integrity of the maleimide imide as a key H-bonding component, there are some recent reports of
N-substitution to achieve non-kinase effects. In 2017, Sun et al. synthesised a number of new BIM compounds of which BMA-155 and its hydrochloride salt (
Figure 12) were identified as potent anticancer compounds operating through the NF-kB p65 pathway and effecting apoptosis both in vitro and in vivo
[78][51]. BMA-155 is modified at the maleimide nitrogen by ethylamine substitution so this template opens up new structural avenues in BIM bioactivity. More recently, in 2022, Kumar et al. generated a series of aminoalkyl-substituted BIMs which incorporate substitution on both indole nitrogens and the maleimide nitrogen
[79][52]. These compounds were reported to preferentially stabilise the G-quadruplexes of
c-MYC and
c-KIT and provide a new starting point for the development of BIM ligands capable of regulating gene expression.
Finally, aside from clinical and pharmacological endpoints, there exist other BIM targets which present opportunities in fields such as sensors
[80][53].
6. X-ray Crystal Structures of Bisindolylmaleimides and Kinases
As seen in
Section 3, the kinase-targeted effects of BIMs can be rationalized through crystal structure formation as ligands. To date, X-ray crystal structures have been solved for BIM compounds in a number of kinases, as detailed in
Table 2. It is clear that BIM compounds are capable of forming crystal structures across the kinase families and, interestingly, the two most often quoted targets of BIMs PKC-β and GSK-3β are in the minority of solved structures. This, however, does not prevent the use of modelling to plot interactions, and indeed high throughput in silico screening has been used extensively in the last 10 years to develop the scope of targets and to rationalize the effects of these potent compounds.
Table 2. Summary of BIM compounds solved as ligands in kinase crystal structures [74,81,82,83,84,85,86,87,88,89,90,91,92,93,94].
BIM-VII |
DMPK1 |
AGC |
2VD5 |
[ | 86 | ] | [ | 59] |
BIM-VIII |
PDK1 |
AGC |
1UVR |
[74][47] |
Ruboxistaurin 61 |
PDK1 |
AGC |
1UU3 |
[74][47] |
PIM1 |
CAMK |
2J2I |
[87][60] |
BfIM 71 |
GSK-3β |
CMGC |
3SD0 |
[88][61] |
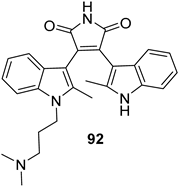 |
PKC-β |
AGC |
2I0E |
[89][62] |
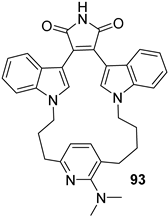 |
GSK-3β |
CMGC |
2OW3 |
[90][63] |
Indolyl phenyl maleimide 94 |
GSK-3β |
CMGC |
1R0E |
[91][64] |
Indolyl pyrimidinyl maleimide 95 |
JAK3 |
TK |
3PJC |
[92][65] |
Indolyl pyrroloquinolinyl maleimide 96 |
MET |
TK |
3RHK |
[93][66] |
Indolyl quinazolinyl maleimide 97 |
PKC-α |
AGC |
3IW4 |
[94][67] |
7. Conclusion
Looking at the starting BIM standards (BIM I-XI), it is clear that PKC kinase and protein kinases in general are noted targets, and so any new BIM derivative should be screened for this activity. It is also evident that multiple targets can be effectively inhibited by compounds with the bisindolylmaleimide pharmacophore and indeed selectivity within the kinases can be achieved, which suggests there is significant value in further development.
Since the early 1990s, both acyclic and macrocyclic BIM agents have excelled during in vitro studies and progressed to in vivo testing and human clinical trials. The introduction of different aryl units to the BIM pharmacophore proved to be a successful strategy to enhance target selectivity. The BIM class offers unique opportunities for drug repurposing due to its known safety profile and indeed many BIMs have completed clinical trials, which is evidence of their real-world impact. Beyond the BIM structures we have investigated, there are many other aryl maleimides [95] and bisindole derivatives [96–98] which have notable bioactivity, and hence this expanding field is sure to maintain real-world relevance in the future.