The World Health Organization (WHO) Global Technical Strategy (GTS) for malaria is elimination by 2030 in 35 countries, while a target of global eradication by 2040 has recently been announced (
https://www.who.int/publications/i/item/WHO-CDS-GMP-2019.10, accessed on 23 June 2023)
[1]. The new affirmation follows from the earlier initiative of eradication launched in 2007 and the malERA initiative in 2011
[2,3][2][3]. The malERA elimination and eradication agenda has highlighted key challenges that need to be overcome to achieve elimination and eradication by the global community. These include (a) Prioritizing how to detect the foci of the burden of disease and drug resistance, and how they spread; (b) Ensuring a clear understanding of the operational process of surveillance and response based on scientific evidence; (c) Ensuring that the biology of
Plasmodium vivax (P. vivax) is well understood and constitutes part of the strategies for elimination in all countries, including those in sub-Saharan Africa; (d) Sustaining capacity building and engagement efforts between scientists, policymakers and local actors so that the focus on elimination and eradication is clear and upheld
[2,3,4][2][3][4].
2. Life Cycle of Human Plasmodium Parasites and Their Recently Discovered Tissue Niches
2.1. Sporozoite Inoculation and Invasion of Tissues
The epidemiology of malaria depends on a complex interplay between the intermediate host, parasite, definitive vectors and the environment
[13,14][5][6]. These factors differ from one geographic region to another, thus the epidemiology also differs accordingly. There are five
Plasmodium species in the phylum
Apicomplexa and order
Haemosporidae that cause malaria in humans:
P. falciparum,
P. vivax,
P. ovale curtisi,
P. ovale wallikeri, (
P. ovale),
P. malariae and
P. knowlesi [15][7]. An infection is initiated when a female
Anopheline mosquito infected with the parasite inoculates an estimated 15–123 sporozoites under the skin of an individual while probing for a blood vessel to take a meal
[16,17,18,19,20][8][9][10][11][12]. While under the skin, the sporozoites get activated based on the environmental milieu, express proteins required for gliding motility and cell traversal and travel through the blood to the liver using the sinusoids as entry points to hepatocytes
[21,22,23,24][13][14][15][16]. The sinusoids are lined with resident macrophages, the Kupffer cells that aid in the transmigration of the sporozoites via gliding motility
[25][17] through the sinusoids to reach hepatocytes
[26,27][18][19]. Cell traversal proteins enable entry through the hepatocytes until one is selected for development; alternatively, the sporozoites may use the lymphatic system to enter tissues with the help of the sinus lining monocyte–macrophage system
[28][20].
2.2. Entry into Extrahepatic Tissues/Cryptic Sites
It has recently been reported that sporozoites can access and develop in extrahepatic tissues of the body, including the spleen, bone marrow and lungs
[17,29,30,31][9][21][22][23]. This raises the question of how the parasites enter these non-hepatic tissues or cryptic sites. In some vertebrates (birds and lizards), sporozoites can enter tissues and differentiate further using the monocyte–macrophage phagocytic system, including in the skin
[25][17]. The Kupffer cells, which form the monocyte–macrophage system in the liver, are deficient in the production of reactive oxygen species (ROS), fail to respond to interferon-gamma during infection, are defective at secreting microbicidal molecules and serve as permissive hosts for intracellular parasites
[32][24]. These characteristics have also been seen in spleen red pulp macrophages
[33][25]. Comparatively, it may be that the culpable cells permitting intracellular parasite migration into non-hepatic tissues are the sinus lining monocytes/macrophages in the bone marrow, Langerhans cells in the skin, and alveolar macrophages in lung cells (
Figure 1). These require further interrogation as they have a bearing on the role of relapses of hypnozoites in
P. vivax and
P. ovale malaria transmission
[31][23] and asymptomatic infections. These could constitute new avenues for blocking malaria transmission using monoclonal antibodies.
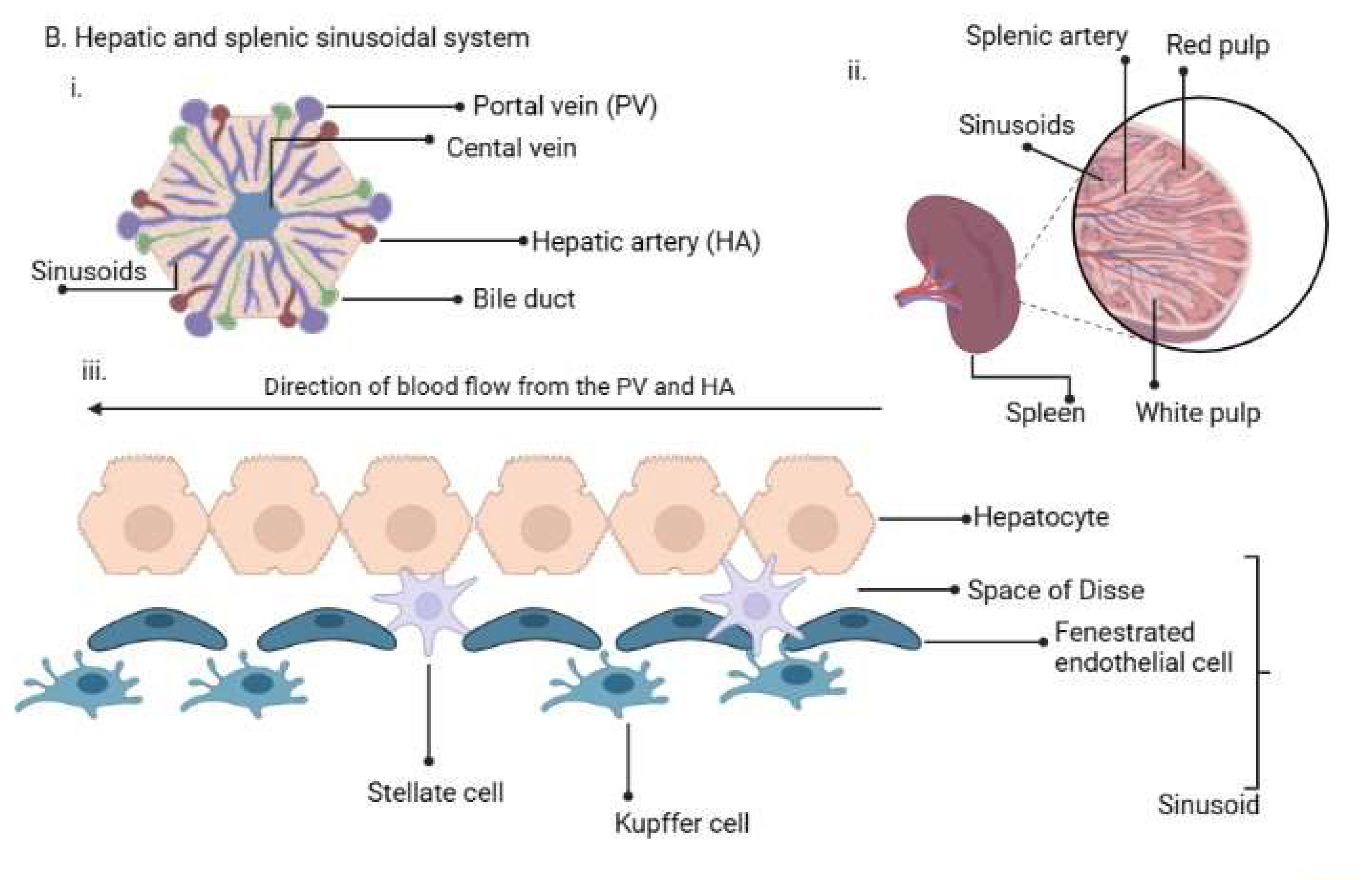
Figure 1. (A). Plasmodium life cycle. (A) Description of the Plasmodium life cycle from the sexual stage oocysts through sporogony to sporozoites, and migration into the salivary gland until inoculation into the human intermediary host during feeding. Sporozoites then migrate in the asexual stage into host tissues and development via schizogony and back to the sexual stages. (B) i. a liver lobule showing the triads, ii. a transverse section of the spleen showing an area of the sinusoid system, and iii. the hepatic sinus lining monocyte–macrophage system (sinusoid) Parasites seeking entry interact with the Kupffer cells and stellate cells through proteoglycans. Cells arrive at the sinusoids from blood flow coming through the intersection of the Portal vein and the Hepatic Artery in the liver. In the spleen, blood flows through the splenic artery into the central artery, either through the perifollicular zone and then through the venous sinusoids in the fast circulation, or the red pulp of the cords (consisting of fibroblasts and reticular fibers, but without endothelial cells) in a slow microcirculation. The slow circulation enables the mononuclear phagocyte system to remove particulate matter and generate the requisite immune response. From the red pulp, the blood squeezes through the venous sinusoids, which filter the blood further removing infected RBCs or intraerythrocytic bodies and exiting through the splenic veins to the portal vein.
2.3. Development of Asexual and Sexual Forms
The development within the tissues is impacted by the specific tissue environment. Rapid transcriptional changes occur in each parasite depending on the tissue milieu that determines the success of transmission
[21][13]. In the liver, the sporozoites grow into liver schizonts that undergo schizogony and transform into merozoites, which are the red blood cell (RBC) infective forms
[34][26]. The merozoites exit hepatocytes through the sinusoids and Kupffer cells to infect RBCs in the form of merosomes, which are merozoites enclosed in a membrane
[35][27]. The merozoites use gliding motility similar to sporozoites for entry into RBCs
[36][28].
In
P. vivax and
P. ovale, some sporozoites cease replicating and enter a dormant hypnozoite stage
[37][29] from which they can relapse when triggered, entering a new replication mode and generating new merozoites
[38][30]. Recent reports reveal that
P. vivax hypnozoites express genes towards the gametocyte stage early in their development and seem programmed to commit to gametocytogenesis upon activation
[39][31]. In this way, they serve as parasite reservoirs for propagation. In the RBCs, merozoites go through early ring forms, late trophozoites and blood schizonts that expand, burst through the RBCs and initiate a new cycle of infection as merozoites (
Figure 1A).
P. vivax merozoites are restricted to the invasion of reticulocytes while
P. falciparum parasites are not restricted, although they show a preference for younger RBCs during invasion
[40][32]. The schizonts contain about 16–32 merozoites
[41][33]. The cycle of RBC invasion by merozoites leads to a paroxysm of fever that repeats every 24 h in
P. knowlesi, 48 h in
P. falciparum,
P. vivax and
P. ovale and 72 h in
P. malariae [41][33]. These paroxysms give the respective descriptors of quotidian, tertian and quartian malaria, respectively, to the disease. During the asexual RBC cycle, some of the merozoites develop into immature male and female gametes called gametocytes, which mature to initiate the sexual cycle
[42][34]. The immature forms, which are not visible in the peripheral circulation, are present in the bone marrow and spleen where they mature and re-enter the circulation
[34][26]. In
P. falciparum, five developmental forms have been observed and characterized as stages 1-V, although only the stage V form is seen in the peripheral circulation
[43][35]. In
P. vivax, however, all the asexual and sexual stages of the parasite can be seen in the peripheral circulation
[44][36]. The mature gametocytes are taken up during feeding by anopheline mosquitoes and develop through gametocytogenesis to produce male microgametes and female macrogametes. The microgametes undergo exflagellation before fertilization with the macrogametes into zygotes
[45][37] in the mosquito midgut (
Figure 1A). Genetic recombination occurs during this period, leading to a diploid genome. The zygotes develop further into motile ookinetes in the midgut. Subsequently, the ookinetes move from the midgut epithelium to the basal lamina and develop into oocysts via a sporogonic cycle. Sporogony continues in the oocysts, generating numerous sporozoites
[46,47][38][39]. Once mature, the sporozoites are released from the oocysts, after rupture, into the hemolymph and migrate into the salivary gland ready for a new cycle of inoculation and infection of humans
[48][40] (
Figure 1A). The genes expressed in the developmental cycle of the parasite appear to be species-specific based on the host and vectors that the parasite interacts with during the life cycle
[48][40]. These differences are very important in the life cycles of
P. vivax and
P. ovale, which have hypnozoite stages. The gene expression pattern in the parasites is modulated based on host factors, allowing early release and viability of the gametocytes, thereby sustaining transmission
[44][36].
P. vivax is also established to adapt very well to climatic variations and multiple vectors, which adds to the difficulty associated with eliminating this parasite
[47,49,50][39][41][42].
All the blood stages of
P. vivax have been observed in the extrahepatic tissues (bone marrow and spleen). The immature gametocytes are enriched in the bone marrow parenchyma and the spleen
[51[43][44][45],
52,53], while the spleen is further enriched with late-stage asexual forms, trophozoites and schizonts
[31][23]. Schizogony in the bone marrow and spleen can also generate merozoites for RBC infection
[31][23]. Schizonts also accumulate in the lungs and adipose tissues
[52,53][44][45]. These extrahepatic sites seem to be reservoirs for transmission and sources of sustenance of transmission, as they are protected from immune attack and drug treatment and occur in asymptomatic individuals
[25,31,54][17][23][46]. Whether these synchronize with the liver stages to release merozoites into circulation is currently unknown.
2.4. The Reticuloendothelial (RES)/Mononuclear Phagocyte System (MPS) in the Plasmodium Life Cycle
The term ‘RES’ was originally coined by Karl Albert Ludwig Aschoff in 1924
[55][47] to reference cells involved in phagocytosis. The
Reticulo referred to the tendency of these large cells to connect via cytoplasmic projections to form a network or reticulum, while
endothelial referred to the closeness to the vascular endothelium. In later years, RES was renamed as cells of the mononuclear phagocyte system (MPS)
[56][48] based on their morphology and kinetics and their ability to phagocytose. Currently, they are made up of three key cells based on their function and phenotype: monocytes, macrophages and dendritic cells
[57][49]. The cells may be resident in their tissues or recruited, lining the sinusoids of their respective tissues. Macrophages are derived from embryonic progenitors, starting from the yolk sac and fetal monocytes and are subsequently distributed throughout the developing tissue through the peripheral circulation
[58,59][50][51]. These cells subsequently self-renew in the absence of adult hematopoiesis
[60][52]. Monocytes, on the other hand, are derived from monocyte progenitor cells and dendritic cells from a dendritic cell precursor (adult hematopoietic stem cell precursors)
[60][52]. Based on this classification, the previously classified RES cells—Kupffer, microglia, alveolar macrophages, splenic red pulp macrophages, Langerhans cells and lymphatic cells—are all considered as belonging to the macrophage type of cells as they are embryonically derived
[60][52] (
Figure 1B). An important point, though, is that while their origins are the same, the gene expression patterns are dependent on the tissues in which they are resident
[61][53]. Interestingly, in all the indicated tissues,
Plasmodium sexual and asexual parasites have been observed as discussed below, but much more studied in
P. vivax.
2.5. RES/MPS as Host Sites of Cryptic Infections by Plasmodium Parasites
Blood and Liver: Sporozoites enter the bloodstream in the peripheral circulation using gliding motility
[36][28], a process that is unique to
Apicomplexan parasites. The parasites stay in the blood until they reach liver sinusoids, where they are initially sequestered. By interacting with heparan sulphate proteoglycans from Kupffer and stellate cells, they enter hepatocytes enclosed in a parasitophorous vacuole of host origin, via gliding motility, through the Kupffer cells
[26,47,62][18][39][54] (
Figure 1B). They finally settle in one hepatocyte to signal an end to the migration and continue to develop into schizonts. It has been suggested that cell entry within a parasitophorous vacuole limits cellular damage during migration, reduces the risk of an inflammatory response to the parasite and is used by the parasite for nutrient intake and efflux of waste materials
[24,63][16][55]. Sinusoids present in tissues of the RES have been reported to harbor sexual and asexual forms of the
Plasmodium parasites following human autopsy studies and rodent experiments
[52,53][44][45]. A question that needs further interrogation is: do
Plasmodium asexual and sexual forms have a preference for the use of sinusoids to enter tissues because of the peculiar role played by macrophages in sinusoid entry?
Bone marrow and the spleen: The bone marrow is a major site for erythropoiesis, while the spleen filters blood to remove old and damaged erythrocytes, particles and pathogens
[64,65][56][57]. The spleen can contribute to erythropoiesis in a time of need, such as blood loss or trauma. The macrophages of the sinusoids and fenestrated endothelial cells play key roles during erythropoiesis and the filtering function of the spleen
[66][58]. Bone marrow macrophages modulate erythropoiesis by close apposition to the developing erythroblasts, while during blood filtration in the spleen, macrophages recognize pathogens using pattern recognition receptors and pathogen-associated molecular patterns and remove them during the open and slow microcirculation through the sinusoids
[67][59]. Parasite entry into the bone marrow and spleen is also suggested to be through gliding motility, like that of leukocytes during their transit to sites of acute inflammation, aided by tissue sentinel macrophages and molecular signals from the pathogens and damaged cells
[68,69,70,71,72][60][61][62][63][64]. Therefore, a role for macrophages assisting in parasite entry, as mentioned previously, would be highly significant in the life cycle of the parasite. It has also been observed that merozoite-infected RBCs could home into the bone marrow and spleen in a receptor-mediated process due to vascular leakages that provide signals for invasion
[53][45]. These observations were seen in both the rodent
P. berghei parasite and the human
P. falciparum, indicating that the bone marrow and spleen are major sites for parasite development during the
Plasmodium life cycle. The unique biology of
P. vivax and
P. ovale, i.e., having hypnozoite stages, presents an even greater intriguing scenario considering that this stage can cause relapse after several years
[73,74,75][65][66][67]. Although malaria relapses are attributed to a hypnozoite source in the liver, the current understanding of the life cycle may point to there being additional sources of cryptic ‘
non-liver rejuvenants’ of dormant parasites
[76][68]. A large proportion (>90%) of chronic
P. vivax infections are asymptomatic, subpatent and submicroscopic
[74][66]. These non-tangible infections have been observed to be present in the spleen and form the largest biomass of
P. vivax infections, accounting for close to 98% of the biomass
[31][23]. In addition,
P. vivax genes involved in sequestration and cytoadherence are spleen-dependent
[77,78][69][70]. Therefore, the spleen and bone marrow, in particular, serve as important sites in the life cycle of
P. vivax. These cryptic infections must be considered carefully in surveillance strategies to ensure that tools for intervention are optimal. The use of passive case detection as currently conducted in most malaria-endemic countries in sub-Saharan Africa, focusing only on
P. falciparum malaria, would fail to lead to malaria elimination.
Lymphatic system: The lymphatic system maintains tissue fluid homeostasis and coordinates the transport of immune cells to tissues
[79][71]. The system harbors asexual
Plasmodium parasites of all stages, including merozoites
[18,80][10][72]. The source of the parasites could be sporozoites at the time of inoculation or directly from infected RBCs in the circulation
[18][10], considering that the lymphatic system is closely linked with the hepatic sinusoids
[81][73]. Parasite development could occur in the lymphatic system, leading to the generation of merozoites that can stay latent for some time before joining the peripheral circulation to invade new RBCs, hence sustaining the life cycle
[80][72]. The subscapular macrophages of the lymph nodes may be the main cells permitting the entry of intracellular pathogens; this has been seen for viruses as well
[82][74].
2.6. Plasmodium vivax Conundrum and Strategic Activities in an Infection
A pathogen’s ability to enable easy uptake by host phagocytes is described as the Trojan Horse model
[83][75]. It appears that cells of the MPS are frequently used by intracellular parasites as Trojan Horses for entry into host tissues
[84][76]. Prolonged environmental changes and interactions between pathogens and host cells are key drivers of adaptation and enablers of the spread or resilience during an infection
[85,86][77][78]. These changes ensure some level of disengagement with or manipulation of the host immune system to ensure survival. The
Plasmodium sporozoite has three main biological characteristics: to be motile, traverse cells and invade host cells
[87][79]. For these functions, the sporozoites and merozoites have characteristic secretory granules: dense granules for modifying host cells, rhoptries for forming the parasitophorous vacuoles and micronemes for RBC invasion
[88][80]. Sporozoites have been shown to contain rhoptries and micronemes, while merozoites have all three granules. Gliding motility is the main enabler of the three characteristics
[88][80]. These characteristics are shared by
Plasmodium parasites.