Nanotechnology pertains to the employment of nanoparticles and furnishes the potential to fabricate novel materials and products possessing improved quality. The nanomaterials may be used as; nanosensors, nanocides, nanofertilizers, nanobarcodes, and nano-remediators, which play a significant role in modern agricultural practices. However, the physical and chemical processes of nanoparticle production is neither economical nor environmentally sustainable. Therefore, the need for green or biogenic nanoparticles obtained from plants, bacteria, fungi or their metabolites has emerged as novel, sustainable, economical, biocompatible, and eco-friendly technology.
1. Introduction
Rapid population growth, extensive rise in anthropogenic activities, and global climate change have resulted in a wide range of contaminants in different environmental matrices and stress-related degradation of soil quality, leading to preponderate decline in crop productivity across the globe
[1]. Abiotic stress is amongst the most alarming issue that is gravely endangering ecosystem stability at a rapid pace
[2]. The most prevalent abiotic factors affecting agricultural productivity include salinity, drought, heat, chilling, and heavy metal toxicity. Various ecologically friendly strategies are being employed to limit the detrimental impact of abiotic stressors and promoting plant stress adaption ability, and dealing with the global issue of environmental pollution. Nanotechnology is a multidomain area covering; chemistry, engineering, biotechnology, microbiology, and physics that function for committed development, enhancement, and use of nanoscale (1–100 nm) structures
[3]. Owing to their minute size and high surface area to volume ratio, nanoparticles (NPs) exhibit novel properties differentiating them from bulk materials, such as physical strength, stability, optical characteristics, reactivity, electrical conductance, and magnetic properties
[4]. Therefore, NPs are employed in diverse fields’ consisting; pharmaceutics, cosmetics, health care, biomedical, textiles, food, optics, electronics, sensors, optical devices, electrochemistry, energy, and agriculture. Nanotechnology has the capacity to revolutionize the agricultural and biomedical sectors by the employment of biosensors, nutraceuticals, genetically modified plants and animals, plant growth regulators/promoters, intelligent drug delivery systems, nanoherbicides, nanopesticides, and nanofertilizers
[3].
The chemical and physical techniques utilized to synthesize NPs include; irradiation, sonication, pyrolysis, laser ablation, and arc discharge. The chemical methods use hazardous reducing compounds, sodium borohydrate and hydrazine, and result in the production of toxic by-products
[5]. The combined energy and capital requirements of these technologies, along with the use of hazardous compounds, nonpolar solvents, synthetic additives, and capping agents, therefore limiting their use in clinical, biomedical, food, environmental, and agricultural applications
[2]. Therefore, the hunt for a safe, dependable, nontoxic, and environmentally acceptable method to create NPs has led researchers to focus on “green” chemistry and bioprocesses
[6]. Plants parts (seed, flower, leaf, bark, peel), bacteria, fungi, actinomycetes, yeast, viruses, and enzymes have been investigated for developing stable and homogeneous NP synthesizing agents at physiological pH, temperature, and pressure in a clean, environmentally sustainable manner. These organisms are prospective biofactories for NP synthesis since they possess the inherent capacity to biosynthesize NPs in intracellular or extracellular environment
[7]. Amino acids, peptides, protein, polysaccharides, tannins, flavonoids, phenols, and vitamins are important reducing and capping agents in biological synthesis and are vital for controlling the size and shape of NPs. The biogenic NP possesses high polydispersity, dimension, and stability. Numerous reports on the applications of biogenic NP in biomedical and clinical sectors are available, however very few studies describe their applicability in the agriculture and environmental sectors
[8][9][8,9].
2. Biogenic Nanoparticles and Their Synthesis
Different chemical methods have been employed for the synthesis of NPs from a wide range of sources. However, in recent times, the inclination towards “green” technologies employing low-cost, renewable, and eco-friendly materials has been observed. The methods of NP synthesis are categorized into top-down and bottom-up approaches (
Figure 1).
Figure 1. Schematic representation of the synthesis of nanoparticles by top-down and bottom-up approaches and their applications.
2.1. Conventional Synthesis Method
The physical and chemical methods are used in a conventional approach. The top-down method consists of physical processes such as arc discharge, irradiation, ultrasonication, pyrolysis, laser ablation, thermal degradation, and pyrolysis. The physical methods employ mechanical pressure, energy, evaporation, and condensation for NP synthesis. However, the polydisperse nature of the synthesized material, production of defects, contamination due to raw materials while milling, higher energy demand, use of radiation, high temperature, high cost, and complex instrument requirement limit these methods [10].
2.2. Green Synthesis Method
Schematic representation of the synthesis of nanoparticles by top-down and bottom-up approaches and their applications.
2.1. Conventional Synthesis Method
The physical and chemical methods are used in a conventional approach. The top-down method consists of physical processes such as arc discharge, irradiation, ultrasonication, pyrolysis, laser ablation, thermal degradation, and pyrolysis. The physical methods employ mechanical pressure, energy, evaporation, and condensation for NP synthesis. However, the polydisperse nature of the synthesized material, production of defects, contamination due to raw materials while milling, higher energy demand, use of radiation, high temperature, high cost, and complex instrument requirement limit these methods [11].
2.2. Green Synthesis Method
The chemical and physical methods result in the degradation of chemical groups, production of toxic by-products, and have high energy demand
[11][10]. Therefore, “green nanotechnology” based on biological machinery for the synthesizing biogenic NPs has been explored recently in a bottom-up approach. Synthesis of bio-based nanomaterials such as nanorods, nanotubes, nanoparticles, and nanowires has been economically evaluated as a valuable alternative to fabricating NPs for diverse applications
[2][7][2,7]. Green synthesis uses biological sources, including plant parts, algae, bacteria, fungus, and biological waste as reducing agents in “bionanofactories” for creating biocompatible nanocomposites through the reduction of metal ions from solutions in a low-energy procedure. Biomolecules are capable of reducing metal ions to uncharged atoms, that, on collision, form stable nuclei through the nucleation process with subsequent repetition of the nucleation to form large particles. Thereafter, stabilization occurs through the coating of the synthesized particles by the different biomolecules.
2.3. Nanoparticle Synthesis by Bacteria
In the microbial-mediated synthesis process, bacterial culture filtrate is employed as a reductant for NP fabrication. Various genera of bacteria have been used previously for NP synthesis;
Pyrobaculum,
Aeromonas,
Escherichia,
Burkholderia,
Stenotrophomonas,
Bacillus,
Klebsiella,
Bhargavaea,
Weissella,
Brevibacterium,
Corynebacterium,
Desulfovibrio,
Enterobacter,
Halomonas,
Lactobacillus,
Listeria,
Pseudomonas,
Plectonemaboryanum,
Rhodobacter,
Rhodococcus, Rhodopseudomonas,
Sphingobacterium,
Streptomyces,
Shewanella,
Staphylococcus, and
Weissella [12][13][14,15]. Microorganisms can synthesize nanomineral crystals, magnetic oxide metals, and nanoparticles extracellularly or intracellularly
[14][16] (
Figure 2).
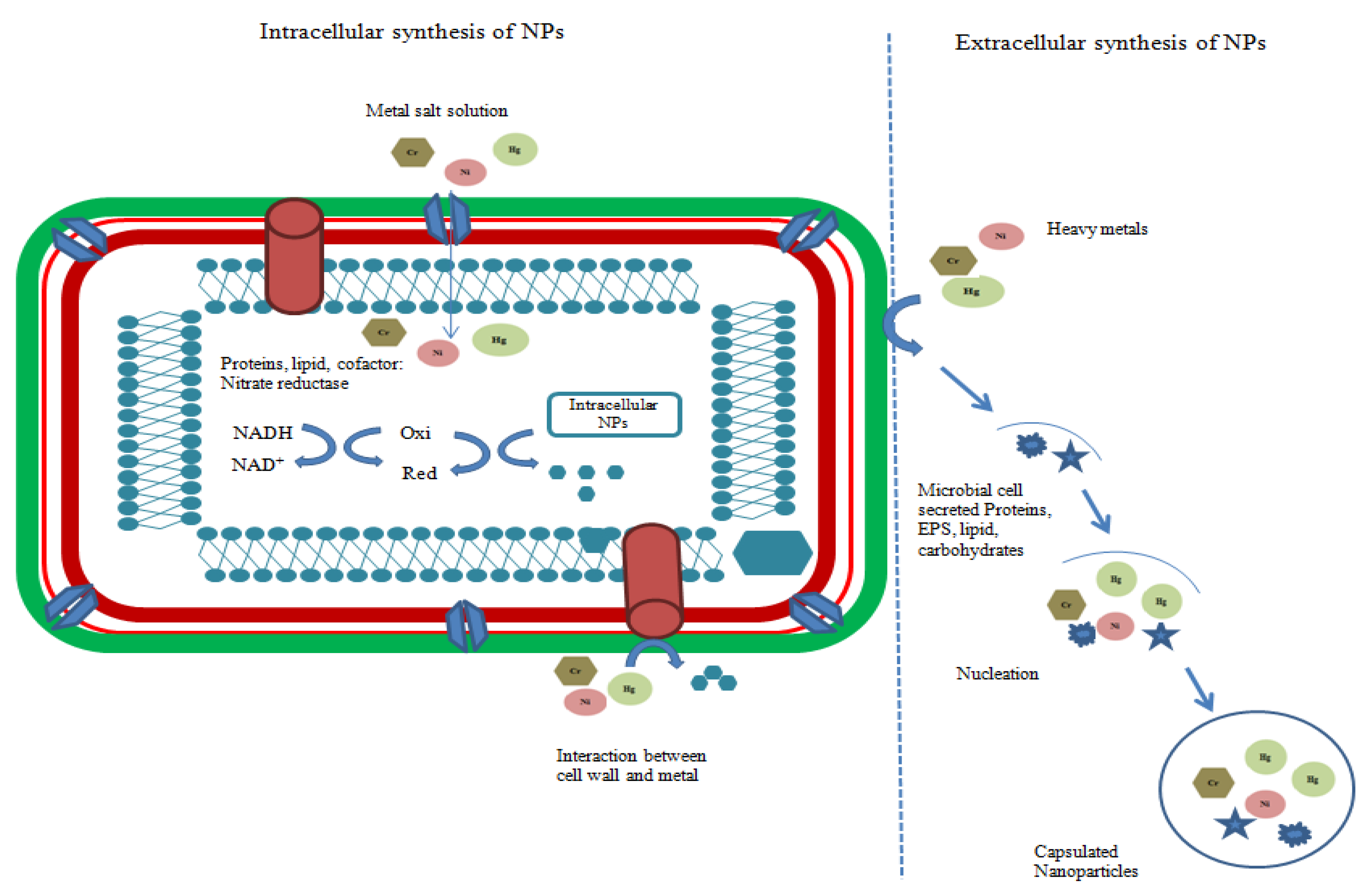
Figure 2. Microbial-mediated biosynthesis of biogenic NPs through intracellular and extracellular modes.
Microbial-mediated biosynthesis of biogenic NPs through intracellular and extracellular modes.
The biochemical processes of microbial-mediated NP synthesis include solubility, adsorption, accumulation, precipitation, toxicity, and efflux
[11][10]. These methods activate the microbial resistance mechanisms for enzyme-mediated cellular detoxification of inorganic particles. Cellular transporters and oxidoreductases, such as nitrate reductase and sulfite reductase, are the major types of enzymes involved
[13][15]. Early reports suggest that NADH/NADPH-dependent nitrate reductases are significant in microbial-mediated metal NP biosynthesis
[15][17].
Bacillus licheniformis synthesizes NADH-dependent nitrate reductase for reducing silver ions to silver NPs
[13][15]. Bacteria convert selenate into red allotropes of Se
0 by selenate and selenite reductases, generally visualized as SeNPs
[16][18]. The charge capping in
Stenotrophomonas maltophilia in an NADPH-dependent reductase enzyme-assisted process results in the conversion of Au
3+ to Au
0 for the synthesis of Au-NPs
[17][19].
In the presence of a higher concentration of heavy metals, microbes generally respond by expressing certain heavy metal resistance genes for NP production. Microbes employ enzymatic reduction, precipitation, complexation, dissimilatory oxidation, and transport vie efflux systems to survive in high metal concentrations. A two-component signal transduction system, BaeSR, is involved in elevated expression of efflux pumps related to metal resistance
[18][21].
Microbes are widely present in the environment, easily cultivable, and show high adaptability in different habitats, making them potent candidates for NP production. However, there are some setbacks of microbial-mediated NP synthesis, such as purification of NP and regulation of geometry owing to limited understanding of biosynthetic mechanisms and scalability. It is difficult to regulate the particle shape and size and produce monodisperse
[7].
2.4. Nanoparticle Synthesis by Fungi
Fungi synthesize a range of enzymes (acetyl xylan esterase, D- glucosidase, cellobiohydrolase, nitrate reductase), peptides, and quinines (naphthoquinones and ansaquinones) that are involved in the conversion of bulk materials into NPs. The amide groups and amino acids with -SH groups are found to undergo a dehydrogenation reaction with metal salts, such as silver nitrate, to form AgNPs
[19][34]. The fungal enzymes, NADH-dependent nitrate reductase and hydrogenase, reduce Ag to Ag-NPs and Pt
+2 to Pt
0, respectively
[15][17]. Phenol oxidases (Mn-peroxidase, laccase, and tyrosinase) reported from xylotrophic fungi are also associated with the oxidoreductase system for NP synthesis
[20][35]. Several fungal genera such as
Aspergillus, Candida,
Fusarium,
Pleurotus, Trichoderma,
Phomopsis,
Phoma,
Penicillium,
Phanerochaete, and
Verticillium assist in metal NP synthesis
[21][36].
2.5. Nanoparticle Synthesis by Algae
Though still in its infancy, phyconanotechnology has emerged as a promising field for the synthesis of NPs from algae in recent times. Algae synthesize different types of secondary metabolites, enzymes, pigments, and proteins that can operate as biofactories for NP biosynthesis. Algae such as
Botrycoccus,
Corallina,
Cystoseira,
Dictyota,
Ecklonia,
Fucuss,
Gracilaria,
Gelidium,
Padina,
Sargassum,
Stereospermum, and
Ulva have been reported for green synthesis of NPs. Ag-NPs produced by
Ulva lactuca were subsequently applied as a photocatalyst for the removal of methyl orange
[22][57]. The FT-IR data of synthesized NPs suggested the involvement of bioactive components, phenolics, amines, and aromatic rings as capping and stabilizing agents in NP synthesis
[22][57]. The capability of algae to assist in the biosynthesis of NPs is due to active components (alginate and laminarin) in algal cell walls.
2.6. Nanoparticle Synthesis by Plants
Although the biogenic NP synthesis is an alternative approach to conventional methods and a wide range of NPs have been synthesized from different micro-flora, there are some drawbacks. These include production cost, sample error, and subservient bulk production
[13][15]. Thus, many studies are ongoing on the employment of plants and their biomass for the fabrication of NPs. The bark, fruit, leaf, latex, peel, seed, stem, shoot, root, phytochemicals, and essential oils are an abundant source of proteins, carbohydrates, enzymes, vitamins, organic acids, flavonoids, phenols, polyphenols, tannins, and terpenoids
[1]. Different plant varieties including
Achillea wilhelmsii,
Aloe Vera,
Azadirachta indica,
Capsicum annuum,
Calendula officinalis,
Camellia sinensis,
Cinnamomum camphora,
Coriandrum sativum,
Cymbopogon flexuosus,
Datura metel,
Gardenia jasminoides,
Gliricidia sepium,
Ipomoea digitata,
Jatropha curcas,
Mentha piperita,
Moringa oleifera,
Paullinia pinnata,
Pelargonium graveolens,
Piper sarmentosum,
Rosa sp.,
Tagetes sp.,
Tamarindus indica,
Terminalia chebula, and
Zingiber officinale are being employed to fabricate NPs. Phytocompounds catalyze the reduction of metal ions to generate zero valent metal ions that result in aggregation to produce metal NPs
[13][15]. The synthesis and stabilization of Ag-NP from cumin occurs through the oxidation of phenolic groups to generate unstable phenoxy radicals which are resonance-stabilized by electronic rearrangements with subsequent reduction of Ag
+ ions and preventing of Ag
0 nuclei from aggregation
[23][58].
3. Characterization of Biogenic Nanoparticles
The size and physico-chemical parameters of NPs are closely related and are crucial for understanding the chemo-physical structure and matrix composition of these materials. Nanoparticle characterization is performed by techniques such as scanning and transmission electron microscopy (SEM, TEM), atomic force microscopy (AFM), dynamic mechanical analysis (DMA), fourier transform infrared spectroscopy (FTIR), X-ray photoelectron spectroscopy (XPS), vibrating-sample magnetometer (VSM), superconducting quantum interference device (SQUID), dynamic light scattering (DLS), NP tracking and analysis (NTA), X-ray powder diffraction (XRD), and UV-Vis spectroscopy
[24][78]. These methods are employed for analyzing parameters such as particle size, pore size, orientation, fractal dimension, dispersion, shape, crystallinity, intercalation, and surface area of nanocomposites
[25][79].
FTIR is used to detect organic functional groups (hydroxyl, carbonyl etc) present on the surface of NPs, while XRD allows the determination of crystal structure and phase identification
[26][80]. The composition, bonding, surface charge, phase, functionalization, chemical state, crystallinity, polarity, and electrochemical nature of NPs can be characterized by these methods
[27][81].
Additionally, DLS and NTA describe the size distribution of the NPs. DLS enables the study of time-dependent oscillations and simultaneous hydrodynamic diameter and polydispersity index measurements as a result of Brownian movement. The magnetic and mechanical properties of synthesized NPs can be determined by VSM, SQUID, and DMA, respectively
[24][78]. For investigating high-sensitivity magnetic parameters, vibrating-sample magnetometer (VSM) and superconducting quantum interference devices (SQUID) are utilized, having respective sensitivities of 10
−6 emu and 10
−10 emu. Tombuloglu et al.
[25][27][28][79,81,85] have employed TEM, SEM, XRD, and VSM for analyzing the morphological, structural, and magnetic properties of NPs. The thermal characteristics of silver NPs synthesized from
Stereospermum binhchauensis and
Jasminum subtriplinerve were investigated by TG/DTA and the results revealed that
Stereospermum binhchauensis Ag NPs crystallized at 315 °C and
Jasminum subtriplinerve Ag NPs at 345 °C, respectively
[29][86].
Figure 3. Methods of characterization of morphological, structural, thermal, and mechanical characteristics of biogenic nanoparticle and their application in agriculture sector
[30][87].
4. Application of Biogenic Nanoparticles in Sustainable Agricultural Practices
4.1. Application of Biogenic NPs as Nanofertilizers
To ensure the sustainable production of food around the world, numerous efforts are being conducted for the development of fertilizers that do not affect the environment and soil fertility in long term usage. Employment of NPs in agriculture fields in the form of nanofertilizers has increased globally to reduce the dependency on chemical fertilizers
[31][89]. Nanofertilizers (NFs) effectively supply micronutrients and are cost-effective alternatives to conventional fertilizers by reducing phytotoxicity and environmental harm associated with chemical fertilizers
[32][90]. Nanofertilizers assist in the precise release of nutrients that are easily absorbed by plants and produce a variety of physiochemical and morphological alterations, improved productivity, and soil fertility. Magnesium hydroxide NPs produced from
Aspergillus niger filtrate promotes seed germination and the development of
Zea mays plants when applied at the concentration of 500 ppm
[33][91]. Biogenic silver NPs (25 mg/L) enhance seed germination, photosynthetic pigments, and phenolic and protein content in tomato plants
[34][92]. Silver NPs can be utilized as an environmentally friendly nanofertilizer at a concentration of 20 ppm (safe dose for the environment and human health)
[35][93]. Biosynthesized silver NPs significantly affected the germination of
Bacopa monnieri seeds, improved the production of proteins and carbohydrates, and decreased the levels of phenols and antioxidant enzymes
[36][94]. Biogenic ZnO-NPs from
Sphagneticola trilobata improved the seed germination and fenugreek growth
[37][95].
4.2. Application of Biogenic NPs as Nanopesticides
The loss of crop productivity due to phytopathogens has a significant effect on the world economy. Pathogenic fungi, which cause more than 70% of plant diseases, are the greatest barriers of agricultural expansion
[38][32]. NPs have been studied for agricultural applications, including germination, plant growth, stress tolerance, and disease prevention. An innovative and highly effective method for controlling disease causing bacteria in crop plants is the usage of NPs as antimicrobials. NPs are well known for their potent nematocidal, fungicidal, and bactericidal properties due to presence of phytocompounds and biocontrol agents
[39][101]. Subdue and Cruiser MAXX are two commercially accessible fungicides made with NPs. Some NPs damage the bacterial cell membranes, DNA replication, membrane potential, ROS metabolism, ATP production, block apoplastic trafficking, and inhibit the toxin production
[40][102]. NPs inhibit the growth of fungal hyphae and sporangia and supress spore germination in fungi. Iron oxide NPs made from
Azidirachta indica have an inhibitory effect on the apple plant pathogens, such as
Alternaria mali and
Diplodia seriata [41][103]. ZnO NPs control the development of
Fusarium oxysporum in tomato plants and act as an antifungal agent
[42][104]. Copper oxide NPs produced from actinomycetes showed antimicrobial potential against
Phythium ultimum and
Alternaria alternate [38][32].
4.3. Applications of NPs in Abiotic Stress Mangament
Biogenic NPs function as potential agents for mitigating plant stress through modification in osmotic pressure, nutritional homeostasis, expression, and activity of vital antioxidative enzymes such as catalase, superoxide dismutase and peroxidase, enzymes, and proteins linked to proline and nitrogen metabolism
[11][10], resulting in enhanced plant growth. Biogenic Fe-NPs synthesized from
Chaetomorpha antennina and coated with citrate compounds reduced drought stress by improving the protective osmolyte concentration and the activity of antioxidant enzymes in
Setaria italica [43][113]. Ahmed et al.
[1] reported that FeO-NPs synthesized from
Bacillus strain (RNT1) increased the nutrient uptake and upregulated the genetic mechanism, assisting in alleviating the toxic impacts of drought and cadmium in rice. Exogenous application of Zn-NPs synthesized from
Sorghum bicolor leaf extract resulted in the induction of antioxidant defense mechanisms and improved
Abelmoschus esculentus growth and photosynthetic rate under salinity stress
[44][114]. Biogenic NPs regulate nutritional homeostasis in plants by providing cell protection under salt stress conditions
[11][10]. Foliar application of plant-based Au-NPs significantly changed the amount of ion accumulation in different plant parts, increased nitrogen metabolism and enzymatic and non-enzymatic antioxidant metabolism (ascorbic acid, glutathione, glutathione peroxidase, glutathione reductase, and SOD), thus reducing the amount of ROS and lipid peroxidation
[11][10].
4.4. Applications of NPs as Nanobiosensors
NPs with distinctive features have also been used to modify existing technologies, including the development of “nanobiosensors”, consisting of biosensors and NPs for enhanced detection capabilities. Nanobiosensors create real-time response signals that are easily collected and analyzed
[45][116]. Different NPs including nanowires, nanotubes, and nanocomposites are used in nanobiosensors for identifying physical and chemical differences, monitoring bioactive chemicals, and quantifying contaminants.
Nanobiosensors can be employed in various ways along the entire agri-food supply chain, including soil condition detection, crop protection, and as diagnostic tools for pest identification during storage and quality assurance
[46][118]. Owing to their capacity to detect processes and identify changes, nanobiosensors have contributed to the development of smart agriculture or precise farming by measuring the quantity of nutrients needed by crops, seeds viability, and shelf-life of fruits
[12][14]. Precise farming provides complete information of field or soil conditions in order to make correct decisions to maximize yield. The rapid analysis and effective management of expensive agrochemicals, abiotic stress, and phytopathogens, all of which cause significant crop losses, are further benefits of smart plant systems. Smart nanobiosensors can accurately carry out the time-consuming task of routine plant monitoring
[12][14]. Electrochemical detection utilizing DNA-based nanobiosensors can be used to find the
Phytophthora palmivora with higher sensitivity that causes black pod in cocoa
[47][119].
Phytoplasma aurantifolia causes witches broom disease in lemon and can be detected using nanobiosensors that employ the quantum dots fluorescence energy transfer phenomena to achieve 100% sensitivity
[48][120]. Nanobiosensors are affixed to the plant’s leaves, where waves of hydrogen peroxide (H
2O
2) signaling are seen. H
2O
2 is a chemical that reacts in plants being utilized subsequently to send signals that enable leaf cells to produce substances that protect from toxicants and predators i.e., insects
[49][121].
4.5. Application of Biogenic Nanoparticles in Bioremediation
Anthropogenic activities like industrial, agricultural, and other activities are mostly responsible for high concentrations of hazardous pollutants such as heavy metals, pesticides, textile dyes, and others
[50][125]. NPs have been employed as adsorbents, immobilizing agents, photocatalytic agents, and filters for the removal of environmental contaminants (
Figure 45).
Figure 45.
Role of green nanotechnology in bioremediation and environmental pollution control.