Generally, chitin deacetylation can be considered as a non-catalytic liquid-solid reaction. The kinetics can be described by the shrinking core model
, which is applicable for the initial time interval (0–30 min) of the reaction. A modification of this model
takes into account the diffusion capacity of the reagent inside the polymer and makes it possible to predict the degree of deacetylation over the entire tim
To explain the features of the kinetics of the chitin deacetylation reaction—namely, the presence of fast and slow deacetylation regions on the kinetic curve, various hypotheses were proposed: the existence of a reverse acetylation reaction
, changes in the morphology of partially deacetylated chitin during washing, the decrease in alkali concentration during deacetylation
, diffusion of hydroxide ions and reaction products and the effect of chitin particle porosity
, the existence of two parallel reactions leading to the formation of different products, the formation of a reaction inhibitor or inactive intermediates (complexes, hydrates), and the effect of hydration (solvation) of reacting particles on their interaction with chitin
.
The observed shape of the deacetylation kinetic curve (
Figure 3) immediately leads to the assumption of the existence of a reversible reaction and the establishment of an equilibrium state in the reaction mixture (
Figure 2). However, as is known from the chemical properties of organic amines, the conditions under which deacetylation is carried out exclude the possibility of the reaction of acetylation of the amino group with sodium acetate.
2.3. Crystallinity of Chitin
It has been suggested that the presence on the kinetic curve (
Figure 3) of areas of fast and slow deacetylation is explained by the presence of amorphous and crystalline
[61] regions in chitin. This hypothesis was put forward in
[55] and continues to be used in various works
[49][62][63]. It is assumed that in the amorphous regions, the deacetylation reaction proceeds rapidly (the first section of the kinetic curve), while in the crystalline regions, the reaction rate is lower due to the slow diffusion of the reacting particles (alkali molecules or hydroxide ions) to the chitin molecules. Therefore, after the complete deacetylation of amorphous regions, the deacetylation rate decreases, and the second section of the kinetic curve appears.
2.4. Diffusion of Alkali
The results of studies of the kinetics of the deacetylation reaction obtained by
1H and
13C NMR spectroscopy
[57][58], indicate that the main role is played by the rate of diffusion of alkali into the solid particles of chitin. Confirmed by data in the literature
[64][65], the observed decrease in the deacetylation rate is mainly ascribed to diffusion limitations. It is assumed that the rate of heterogeneous deacetylation can be controlled by the effective diffusivity of OH
− through the reacted (deacetylated) layer inside the polysaccharide particles
[53]. According to the model proposed in
[54], the remarkable decrease of the deacetylation rate is attributed to a strong reduction in the diffusivity of the reactant within the polymer preventing the degree of deacetylation reaching 100%, even in the presence of excess NaOH.
2.5. Porosity of Chitin
The porosity of chitin is due to the structure of the shell of crustaceans
[66]. In order to establish the effect of the porosity of chitin particles on the kinetics of deacetylation, scholars studied the deacetylation of chitin with different supramolecular structures—the pore volume, specific surface area, pore diameter, and crystallinity
[46][67]. When dried in air with a gradual evaporation of water, flexible chitin fibrils approached each other, which led to the disappearance of small pores. During freeze-drying, the primary structure of chitin is preserved, therefore, chitin samples dried differently differ in pore size.
3. On the Mechanism of the Chitin Deacetylation Reaction
3.1. The Reaction Mechanism in the First Section of the Kinetic Curve
When considering the mechanism that determines the course of the reaction in the first section of the kinetic curve, special attention is paid to the effect of the hydration (solvation) of particles, the formation of an active particle that causes deacetylation, and the influence of the nature and concentration of alkali on the reaction rate.
3.1.1. Hydration of Chitin/Chitosan and Sodium Hydroxide
The most probable factor affecting the kinetics of the deacetylation reaction is the formation of hydrate (solvate) complexes as a result of the interaction of chitin/chitosan macromolecules and sodium hydroxide with a solvent (water). It was shown in
[46] that the rate of deacetylation of wet (previously kept in water) chitin is significantly lower than the rate of deacetylation of the initial dry chitin. Apparently, the hydration of chitin macromolecules leads to a decrease in the reaction rate in the first part of the kinetic curve.
3.1.2. Alkali Concentration and the Nature of the Active Particle
It has been reported in many studies that the rate of the deacetylation reaction increases with increasing NaOH concentration
[63][64][68], but not monotonously. The degree of deacetylation sharply increases starting from a NaOH concentration of 20–25 wt.%. In this concentration range, there is also a maximum specific electrical conductivity (s) of sodium hydroxide solution. These experimental data can be explained by the complete hydration of sodium hydroxide ions, in which water molecules bind into stable hydrate complexes of cations and anions, leading to a decrease in the number of “free” water molecules.
2.1.3. The Nature of Alkali and the Number of Hydroxide Ions Hydration
The effect of hydration of alkali ions on the kinetics of the deacetylation reaction is confirmed by experiments using NaOH and KOH solutions. The first assumption is that in the case of different cations (Na
+ or K
+) with different ion diameters (d
i) and different hydration energies (G
h), the concentration of “free” water in the system will differ. The hydration energy and size of the K
+ ion are G
h = 398 kJ/mol and d
i = 0.102 nm; for Na
+, G
h = 271 kJ/mol and d
i = 0.138 nm
[69][70]. This difference should affect the degree of hydration of hydroxide ions competing with cations for water molecules in the hydration shell and, consequently, the concentration of “free” water, the reaction rate, and the shape of the chitin deacetylation kinetic curve.
To elucidate the mechanism of the deacetylation reaction in the first section of the kinetic curve, scholars expressed the alkali content not as a percentage or molar concentration, but as the ratio of water molecules to one ion of the dissolved electrolyte. Knowing the molar concentration of alkali (Cal) and water (Cw), it is possible to calculate the number of water molecules per electrolyte ion Cw/Cal (Table 1). In this case, almost identical dependences of the deacetylation reaction rate on Cw/Cal are obtained (
Figure 4).
Figure 4. Dependence of the rate of the deacetylation reaction in the first section of the kinetic curve during the deacetylation time of 0–10 min at 100 oC in a solution of NaOH (1) and KOH (2) on the number of water molecules per one ion of dissolved hydroxide. Original figure.
According to the data, the deacetylation reaction begins to proceed at a noticeable rate when the number of water molecules per electrolyte ion (OH–) is less than that required for complete hydration—less than 6. Before LCH, OH– ions have complete hydration shells and are practically inactive. Upon crossing the LCH, the hydration shell is depleted, and the chitin deacetylation reaction begins. Thus, the reactivity of hydroxide ions appears at the LCH point and further increases with a decrease in the degree of hydration (
Figure 4).
When discussing the mechanism of deacetylation, one should also take into account the fact that the number of ion pairs and associates of alkali molecules increases in concentrated solutions [93,99], which leads to a decrease in the concentration of “free” hydroxide ions. Thus, the actual concentration of hydroxide ions after the LCH point decreases. Consequently, the increase in the activity of hydroxide ions turns out to be greater than that observed in experiments. A similar conclusion was made in a monograph [100], where it was shown that the dependence of the activity of hydroxide ions on the water concentration is approximately 1/[H2O]2. The graph of the dependence of the activity of OH− ions on the molar concentration of water is similar to the dependence obtained (
Figure 4). Thus, water does not participate in the chitin deacetylation reaction but, on the contrary, inhibits the reaction.
3.2. Reaction Mechanism in the Second Section of the Kinetic Curve: Inhibition of the Deacetylation Reaction
The rate of the chitin deacetylation reaction in the second section decreases as shown in many studies
[47][63][71][72][73], and the kinetic curve runs almost parallel to the abscissa axis (
Figure 3). As the alkali concentration increases, the reaction rate decreases. As it was determined in
[43], the activation energy of this step is about 48.76 kJ/mol as it.
It can be assumed that the deceleration of the deacetylation reaction is explained by the formation of a certain amount of water during the reaction. It hypothesized that this water, which is formed in close proximity to the chitin molecule, leads to chitin hydration, reducing the rate of further deacetylation. To determine the source of “free” water during deacetylation, consider the mechanism of the reaction of deacetylation.
The reaction of deacetylation—the reaction of alkaline hydrolysis of acetamide bonds (deacetylation reaction) is a bimolecular second-order nucleophilic substitution of SN
2, in which a strong hydroxyl ion nucleophile attacks the acetamide bond
[69][74]. As a result of the overall deacetylation reaction, no water is produced, and none is consumed. The detailed description of the mechanism includes several steps. In alkali solutions, when the amide carbon is attacked by a hydroxide ion (it can be conventionally called the “first hydroxide ion”), an anionic tetrahedral intermediate (T
O−) is formed
[75][76][77][78]. The reaction is reversible; therefore, the resulting intermediate can either turn into the starting compound or decompose into hydrolysis products. Decomposition pathways may include interaction with various forms in solution that transfer a proton from the hydroxide ion OH
− to the amide ion NHR
−. In dilute solutions, water is postulated as a proton carrier
[77].
At a high alkali concentration, the H
+ proton is taken away by the “second” hydroxide ion OH
− to form a water molecule, and the second intermediate (T
O2−) is formed, which then decomposes to form an acetate ion and an amide ion. The amide ion NHR
−, obtained in this way, takes away the H
+ proton from the water molecule
[76]. The described mechanism of cleavage of the acetamide bond in a concentrated alkali solution can be depicted by the scheme in
Figure 5, where R is the residue of the chitin/chitosan molecule.
Figure 5. Scheme of the mechanism of cleavage of the acetamide bond in a concentrated alkali solution.
As a result, a certain amount of water is released, which locally leads to the hydration of the chitin macromolecule. The experiments showed that the rate of chitin deacetylation decreases with its hydration. In a local area near the surface of chitin particles, water and an acetate ion are formed, and hydroxide ions with sodium cations remain present in the solution (
Figure 6). Thus, in accordance with the proposed scheme (
Figure 6), the reaction can stop in the second part of the kinetic curve as a result of the formation of “free” water, which creates a hydration shell of the chitin molecule and slows down deacetylation.
Figure 6. Competitive mechanism of hydration of reaction particles in the local region of the deacetylation reaction. (
a) Reactions; (
b), (
c)—scheme of hydration of reaction particles. CN—chitin; CS—chitosan; Ac
−—acetate ion; OH
−—hydroxide ion. Original figure.
Formation of Quasi-Stable Chitosan Amide Anion
The second hypothesis, which explains the deceleration of the deacetylation reaction in the second part of the kinetic curve, suggests the formation of a quasi-stable chitosan anion. It results from the cleavage mechanism of the acetamide bond (
Figure 5).
The amide anion of chitosan NHR
− is likely to be stabilized in a concentrated alkali solution. In this case, an excess negative charge accumulates on the deacetylated chitin units, which will electrostatically repel hydroxide ions and reduce the deacetylation rate. If this anion is sufficiently stable, then the deacetylation reaction may stop; this is evidenced by the almost horizontal appearance of the kinetic curve in the second section (
Figure 3) at a high concentration of NaOH. At a lower concentration of alkali, the charge of the amide anion is neutralized as a result of the addition of a proton from a water molecule (as a result of hydrolysis). In this case, the negative charge on the chitin molecule will decrease, and the rate of the deacetylation reaction will increase. These transformations can be represented by the following scheme (
Figure 7).
Figure 7. Scheme of (
a) formation of the amide anion of chitosan NHR- and (
b) its hydrolysis.
At a high alkali concentration, “free” water is practically absent, and the chitosan anion “borrows” a water molecule from hydrated electrolyte ions. With an increase in the concentration of alkali, that is, with a decrease in the degree of hydration of alkali ions, the binding energy of alkali ions with water molecules increases, therefore, the probability of detachment of a water molecule by the chitosan anion decreases. Thus, the lifetime of the chitosan anion will increase. Such a quasi-stable anion creates an electrostatic obstacle for the hydroxide ion to attack chitin molecules, which acquire a negative charge. As a result, the rate of the deacetylation reaction decreases, and the more it decreases, the higher the alkali concentration.
The quasi-stable amide anion of chitosan is destroyed by water. Therefore, after washing the intermediate, the deacetylation starts again. Since the hydration of chitin slows down the reaction rate in the first site, in order to rapidly deacetylate, it is necessary to dry the washed intermediate.
Chitin molecules during the deacetylation reaction under heterogeneous conditions are in an ordered state, as a result of the formation of hydrogen bonds between them
[79][80]. Under such conditions, the negative charge on the amide group of one polysaccharide molecule can interact with the carbon atom in the acetamide group of the neighbouring molecule, on which there is a partial positive charge (
Figure 8).
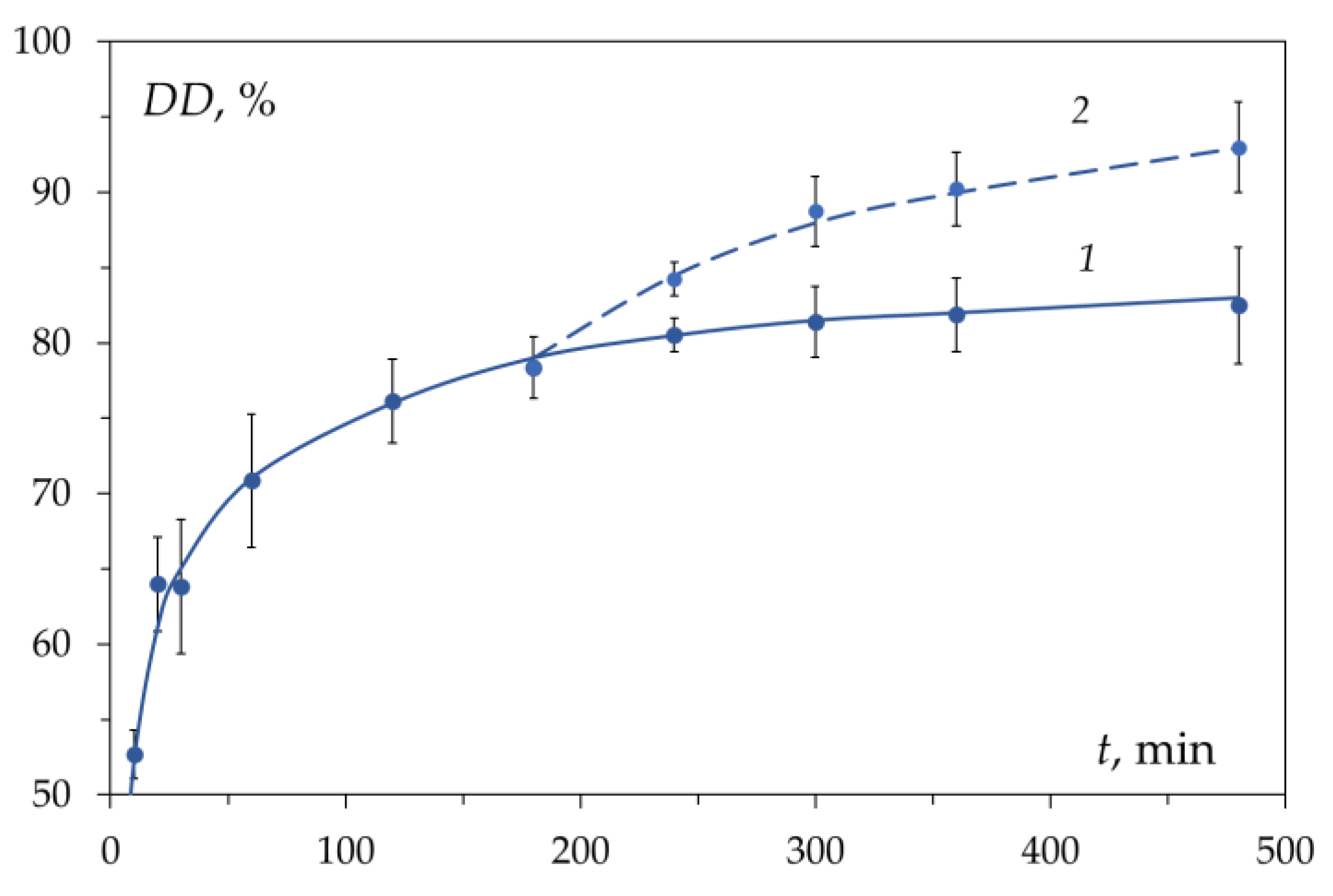
Figure 9. The kinetic curve of chitin deacetylation in 50 wt.% NaOH solution at T = 100 °C without added water (1) and with the addition of water (2) 3 h after the start of the reaction to a NaOH concentration of 40 wt.%. Original figure.
4. Conclusions
This entry considers, in detail, the kinetic features of the reaction of heterogeneous deacetylation of chitin in concentrated (50 wt.%) alkali solutions and analyzes the reasons for the inhibition of the reaction, which make it impossible to obtain chitosan with a limiting degree of deacetylation of 100% as a product of this reaction. The dependence of the degree of deacetylation on the reaction time has a characteristic shape consisting of two sections. The first site (or site of fast deacetylation) is characterized by a sharp increase in the degree of deacetylation within about 30 min from the start of the reaction. The second site (or the site of slow deacetylation) is characterized by a slow increase in degree of deacetylation due to a sharp decrease in the reaction rate. After 3–4 h of reaction time, the maximum achievable value for the degree of chitosan deacetylation, equal to about (80–85)%, is reached.
A mechanism for the reaction of chitin deacetylation is proposed, which explains the features of the reaction kinetics. An analysis of publications on the decrease in the rate of the deacetylation reaction was carried out, and some new experimental results were presented, confirming the conclusions of the scholars. It has been shown that water present in the reaction mixture has the greatest effect on the kinetics of chitin deacetylation, and it can lead to hydration of both chitin/chitosan macromolecules and alkali ions. Within the framework of the mechanism under consideration, a hypothesis is proposed according to which there is a dynamic equilibrium in the reaction mixture between hydrated alkali ions, chitin molecules, and the resulting acetate ions, which can shift depending on the concentration of the reacting particles.
It has been suggested that in highly concentrated aqueous solutions of alkalis, water is almost completely in the form of hydration shells of alkali ions and, to a lesser extent, in the form of “free” water. Apparently, the rate-limiting step in the deacetylation reaction is the hydration of chitin macromolecules by water molecules, that are released during the nucleophilic substitution of acetyl radicals associated with the amino groups of chitins by hydroxyl ions. The acetate ion is the product of the deacetylation reaction. The hydration energy of the acetate ion is less than the hydration energy of the hydroxide ion, so the acetate ion is less hydrated, resulting in some “free” water being released. Under these conditions, hydration of chitin macromolecules occurs in a local region around chitin molecules, where a local high concentration of water is created after deacetylation. The concentration of alkali in the entire reaction volume remains almost constant.
Establishing the mechanism of the chitin deacetylation reaction is of practical importance for the development of technologies for the production of chitosan, and it also makes a theoretical contribution to the understanding of the role of the solvent and hydration processes when considering the kinetics of heterogeneous reactions.